Geology of the Jemez Area, Chapter 12: The Recent Past
The table of contents may be found here.
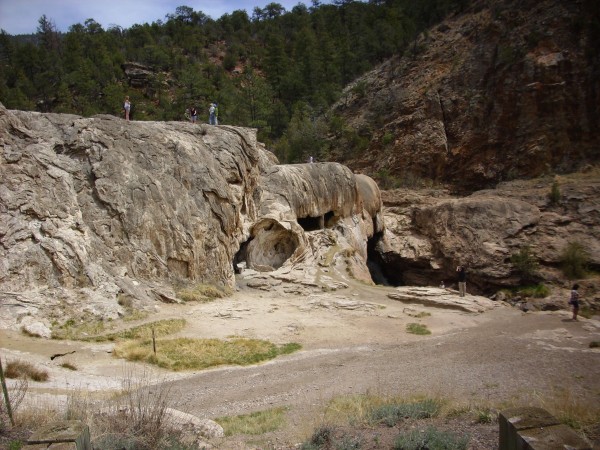
Soda Dam. Looking east from 35.792N
106.687W
The resurgence of the Valles caldera and the eruption of the
ring domes was not the end of geological history in the Jemez.
Geology is an ongoing process. In this chapter, we will look at
geological processes in the last half million years — the very
recent past, in geologic terms.
Potassium-argon dating becomes inaccurate for igneous rocks
younger than about 100,000 years in age, because there isn't time
for enough argon to accumulate to permit an accurate measurement.
Carbon-14 dating is accurate only for ages of less than about
50,000 years. Other methods of dating are inherently uncertain. As
a result, events occurring between 100,000 and 50,000 years ago
were long difficult to date accurately. Fortunately,
high-precision argon-argon dating has begun to close this gap.
An important source of information on life during this time
period is caves, where animal and plant remains are preserved by
cool temperatures and dry conditions away from sunlight. Such
remains are not actually considered fossils, since there has not
been time for significant mineralization to take place. Little of
the original organic material has been replaced by minerals
carried by groundwater.
- Brrr!
- A final burst of volcanism
- The El Cajete Pumice
- The Battleship Rock Ignimbrite
- The Banco Bonito Flow
- El Cajete Lake
- Hydrothermal Activity
- Sulfur and volcanoes
- Hydrothermal alteration
- Erosional landforms
- Weathering and soil
- Desert soils
- Fire
- Colluvium
- Landslides
- Toreva blocks in White Rock
Canyon
- Borrego Mesa
- San Miguel Mountains
- Vallecitos de los Indios
- Alluvial fans
- A river runs through it
- Zeolite ledges
- The Sierra Ladrones Formation
- Terrace gravels
- Alluvium
- Sheetwash
Brrr!
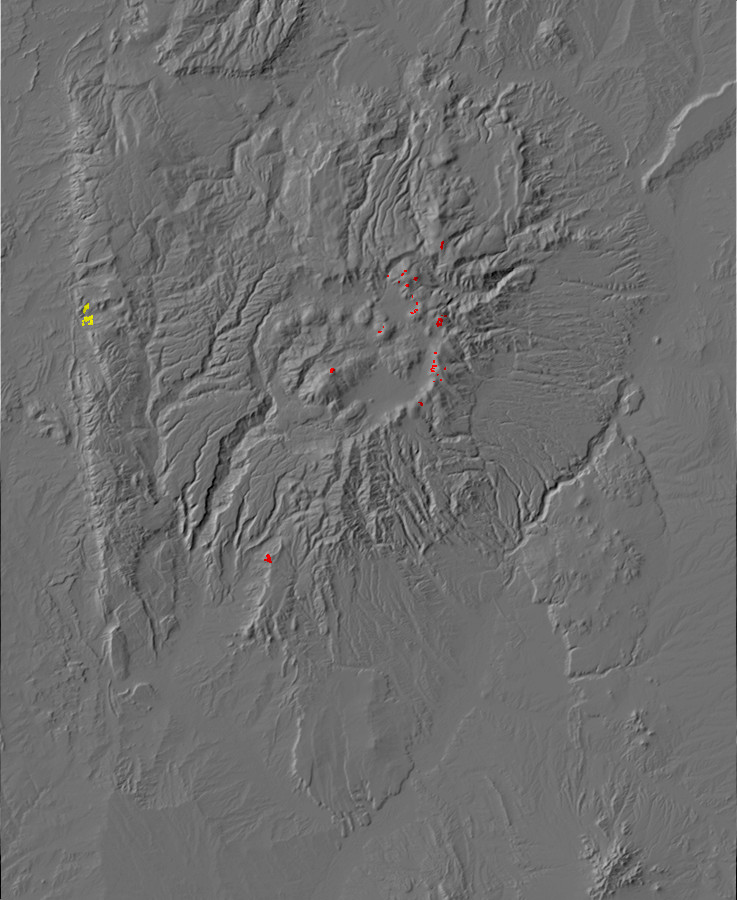
Relief map of the Jemez with suspected rock glaciers
highlighted in red and Shinarump lag field in yellow.
The geological record indicates that the Earth's climate is
usually mild and stable. However, there have been several
intervals of geological time when the climate became unstable,
with large ice sheets developing near the poles and periodically
advancing over much of the temperate zone. These intervals of
geological time are known as ice ages or glaciations.
There have been at least five ice ages during the history of the
Earth. Each occurred when the balance between heat arriving from
the Sun and heat radiated away from the Earth was upset. Since
astronomers have concluded that the Sun has steadily grown
brighter over the last 4.5 billion years, decreases in the solar
input are not a likely cause of ice ages (though there is evidence
that shorter cold spells may be due to changes in solar activity.)
However, the motion of the continents can affect solar heating,
because continents reflect more of the Sun's radiation into space
than oceans do. When continents are covered by epicontinental
seas, this effect is reduced. It is also reduced when continents
drift away from the equator, where most of the solar radiation
falls. But the most likely cause of ice ages is reduced levels of
greenhouse gases, such as carbon dioxide, in the
atmosphere. Carbon dioxide is a greenhouse gas; it lets the
relatively short-wavelength radiation from the Sun pass through to
heat the Earth, but traps the longer-wavelength radiation
re-emitted by the Earth. Carbon dioxide levels increase when
volcanic activity is high or when vegetation dies off and
decomposes. It is reduced when vegetation flourishes or when large
areas of fresh silicate rock are exposed at the surface, allowing
carbon dioxide to combine with sodium, calcium, and potassium in
feldspar. Geologists estimate that weathering accounts for 80% of
carbon dioxide that has been removed from the atmosphere, while
coal and other carbon deposits from vegetation are a mere 20%.
The first known ice age occurred around 2.3 billion years ago, in
the Paleoproterozoic, and likely was caused by reduced methane
levels from the proliferation of cyanobacteria. Methane is an even
more powerful greenhouse gas than carbon dioxide, but the methane
in the early atmosphere was oxidized to carbon dioxide by oxygen
generated by photosynthesis. The second ice age occurred in the
Neoproterozoic, 770 to 590 million years ago, and was the worst
ice age the Earth has experienced. It was likely caused by
continuing decline in carbon dioxide levels and the movement of
the continents towards the equators. This Varangian glaciation, as
it is called, may have covered almost the entire earth in an ice
sheet -- the "snowball Earth." When the ice finally retreated, an
explosion of new forms of life took place, marking the beginning
of the Phanerozoic Era. Two less spectacular ice ages occurred in
the Ordovician-Silurian time interval, 480 to 420 million years
ago, and in the Carboniferous-Permian time interval, 330 to
250 million years ago.
All these ice ages were characterized by periodic advances and
retreats of the ice sheets. This is probably because the advance
of the ice reduced vegetation cover and allowed carbon dioxide to
build up and trap more heat. When the ice retreated, vegetation
began proliferating again and removed the carbon dioxide from the
atmosphere, allowing the climate to cool again. Each advance is
called a glacial period or glaciation and the
intervening warm spells are called interglacials.
We are living in an ice age now, likely triggered by the
collision of the subcontinent of India with Asia and the exposure
of vast amounts of fresh silicate rock in the Himalayan Mountains.
Beginning about 2.6 million years ago, which marks the beginning
of the current Quaternary period, ice began building up in
Antarctica, which has remained mostly covered with an ice sheet
ever since. Certain species of fossil algae called discoasters
became extinct and, almost simultaneously, the first modern horses
appeared, and this moment in time is formally defined as the start
of the Pleistocene, the final epoch preceding our modern Holocene
epoch. Greenland soon iced over as well, and there have been at
least fourteen distinct glacial periods since then, each lasting
about 100,000 years. These were separated by relatively brief
interglacial periods of warmer weather in which the ice sheets
retreated. We are living in such an interglacial period today, the
Holocene, which began 11,700 years ago. Estimates of when the next
glacial period will occur range from within centuries to over
50,000 years, depending on various assumptions about the causes of
glacial periods (which are still uncertain) and the amount of
carbon dioxide that will be released into the atmosphere by
ourselves and our descendants.
Sea level today is somewhat higher than has been typical of the
Cenozoic. All of written human history has taken place during an interglacial,
a period of relative warmth between glaciations. The oceans today
are high enough to flood the margins of the continents, forming
continental shelves under shallow water in the North Sea, the
Bering Strait, and elsewhere. Shallow seas covering continental
crust are called epicontinental seas. The
continents were almost entirely above water, with very little
submerged continental shelf, during the peaks of the ice ages. But
even the continental shelves of today are small compared with what
they have been in the geological past: The Cenozoic has been a
period of unusually low sea levels.
The Quaternary ice age is the culmination of a long period of
global cooling that commenced in the Oligocene, 34 million years
ago, when South America and Australia rifted away from Antarctica
and turned that continent into an ice box. This has led some
geologists to identify the Quaternary Ice Age as merely the
culmination of a much longer Cenozoic Ice Age.
During the Quaternary glacial periods, ice sheets spread over
much of North America and had a profound effect in shaping the
topography of the northern and more mountainous parts of the
continent. The Great Lakes were produced by glaciers, as was Long
Island. However, ice sheets did not extend as far south as the
Jemez Mountains, and there is little evidence that large glaciers
ever formed there. Valleys in the Jemez generally have the V-shape
characteristic of valleys cut by rivers rather than the
distinctive U-shape of valleys cut by glaciers.
However, there are several boulder fields in the higher terrain
of the Jemez that have been interpreted as inactive rock
glaciers. Rock glaciers form when a boulder field is
saturated with water which freezes to ice, and the ice does not
completely melt during the summer months. The outer layer of
boulders acts to insulate the ice. Such permanent ice is called permafrost.
The ice accumulates within the boulder field to the point where
the field becomes ductile, and the mixture of rock and ice can
slowly flow downhill. Current conditions in the Jemez are much too
warm to permit permafrost, even at the maximum elevations of
around 3400 meters (11,000'), but temperatures were significantly
lower during past glacial periods and may have been conducive to
permafrost.
One of the likeliest candidates for an inactive rock glacier is
located on the
west flank of Cerros del Abrigo.
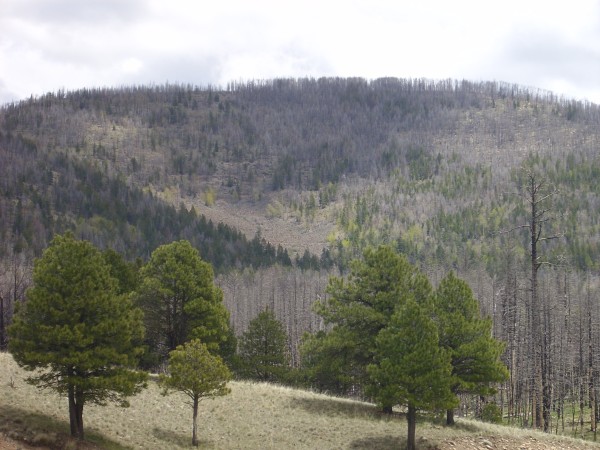
Rock glacier. Looking east from 35
55.928N 106 30.163W
This boulder field shows definite flow features in the satellite
photograph. It is also quite striking at close range.
For one thing, the toe of the field is quite steep.
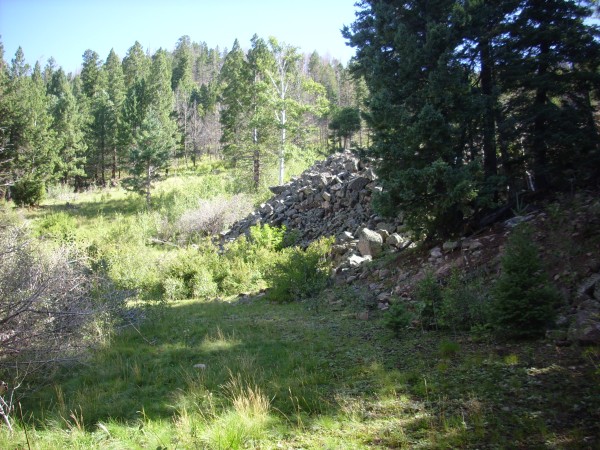
Toe of possible rock glacier. 35
55.867N 106 29.344W
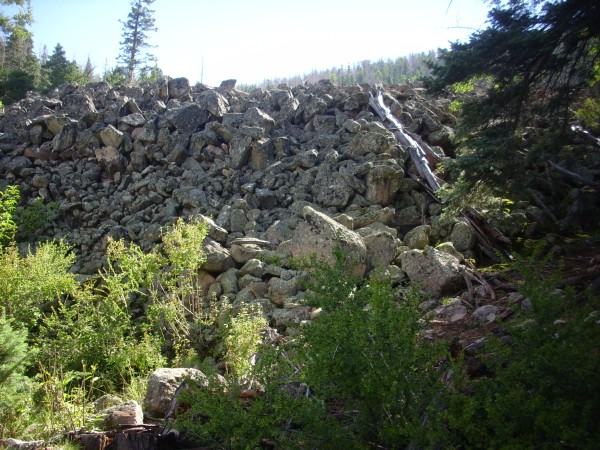
The flanks likewise are remarkably steep.
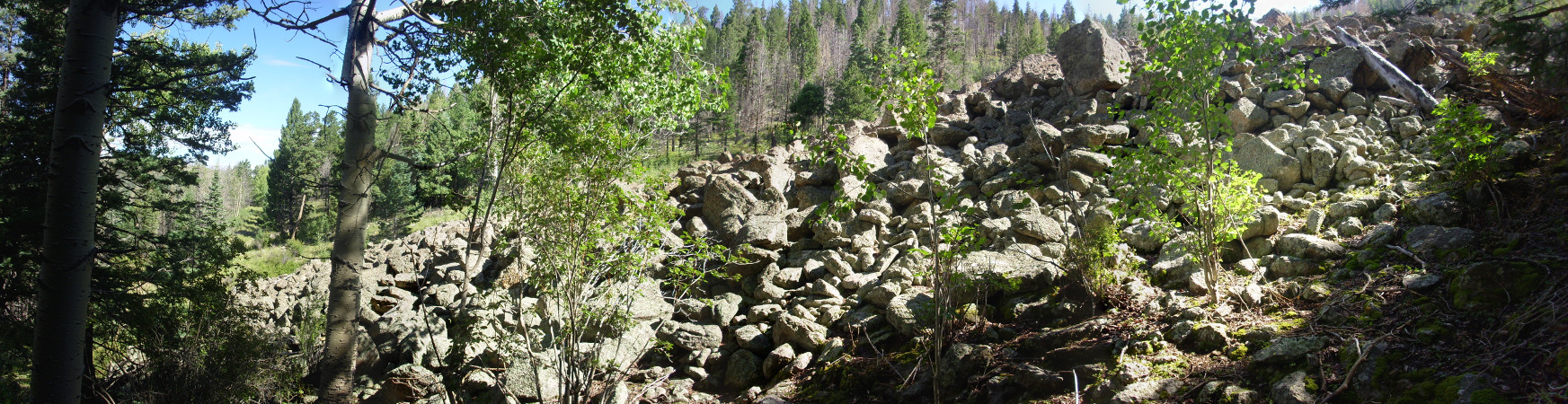
Flank of possible rock glacier. 35
55.850N 106 29.316W
Particularly striking is the smooth slope of the hillside on
which this glacier sits. There are no scattered boulders beyond a
few feet of the edge of the flow.
Views from atop the boulder field:
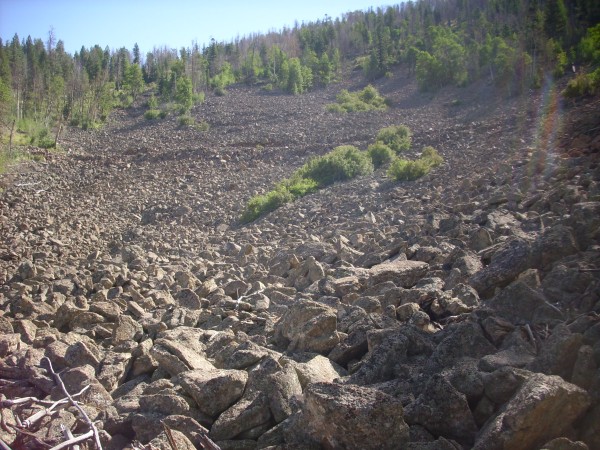
35
55.864N 106 29.302W
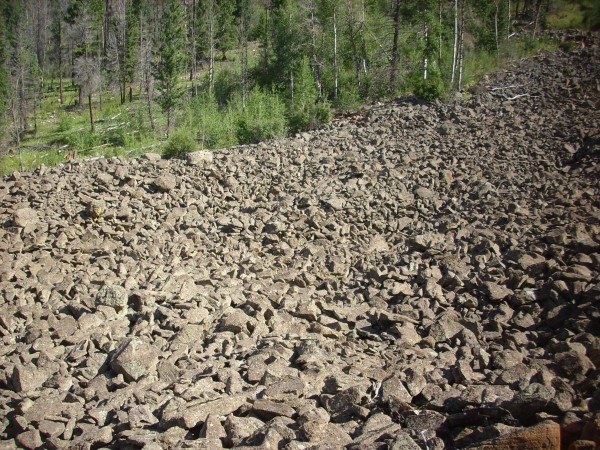
The pictures don’t quite convey the bowl shape of the glacier
surface. One gets the impression that the center was significantly
deflated when the ice melted away, which is probably about right.
The rocks are covered with lichen. This suggests the flow has not
been active in a very long time. There is actually such a thing as
lichenometric dating, based on the diameters of individual
lichen colonies, though it’s very uncertain. But one can make a
guess that the flow has not moved in at least two thousand years.
I did notice one patch at the south edge that looked very fresh.
This small area had either moved recently, or a forest fire burned
off the lichen. Some of the boulders were spalled, as if they had
been subjected to fire.
You can see a logging road crossing the flow in the upper
photograph. Here’s a panorama looking west from the edge of that
road.
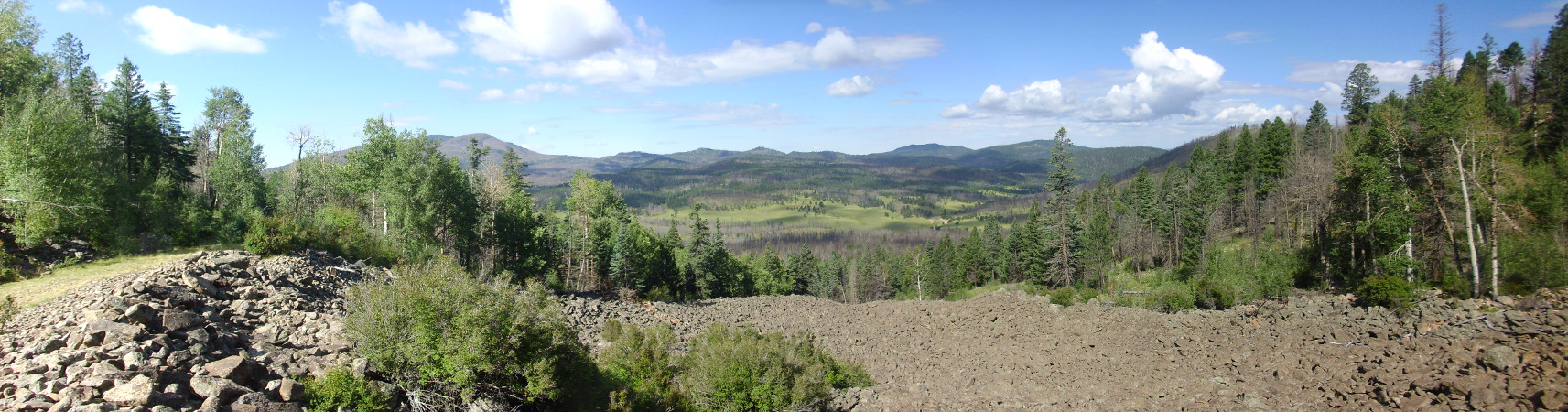
Rock glacier. 35
55.878N 106 29.236W
Behind the trees at left is Rendondo Peak and Redondito. At
center is the rugged terrain of debris flows, megabreccia, and
low-volume Deer Canyon Member flows northeast of Redondo Peak. At
far right are some of the other ring domes of the Valles caldera.
For comparison, here is an active rock glacier on the flank of
Mt. Lincoln, near Alma, Colorado.
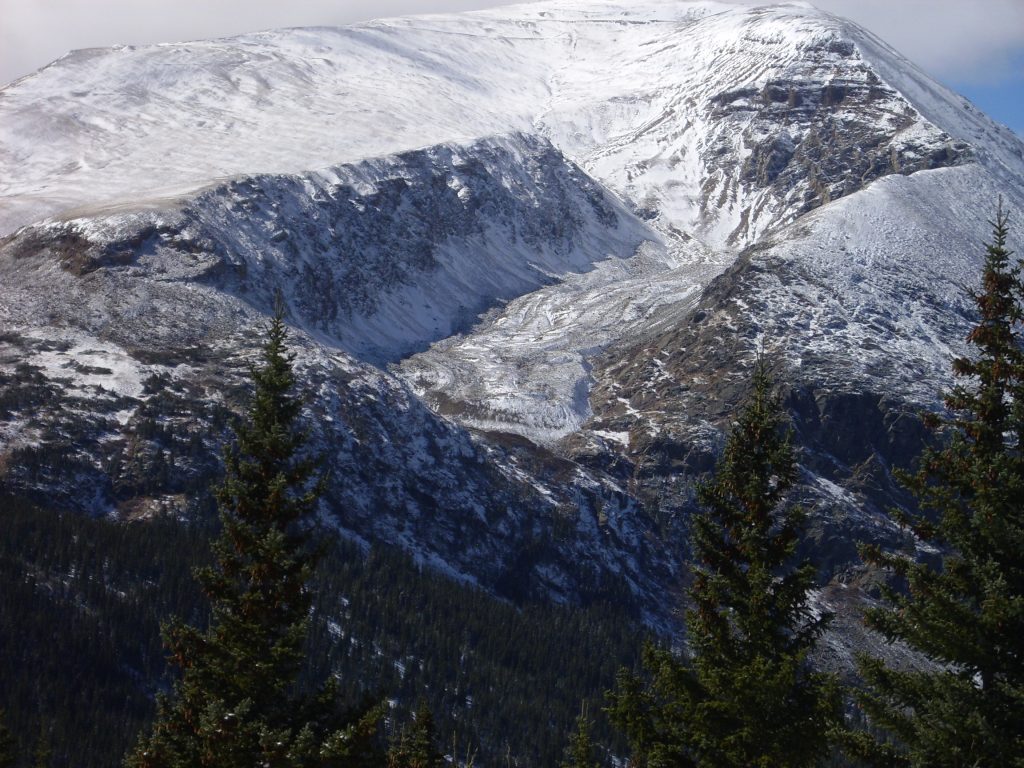
Active rock glacier on Mt. Lincoln,
Colorado. Looking west from 39
21.345N 1063.717W
The rock glacier sits in a hanging valley, which is a result of
conventional glacier activity. During the height of the last
glaciation, a glacier ran down this valley and joined a
larger glacier in the foreground valley. Now the valley is
occupied by the rock glacier, which has the same characteristic
steep front as the inactive rock glacier on Cerros del Abrigos.
With its steep front and flanks, its lack of any matrix between
the boulders, and the indications of pressure ridges in the
satellite photograph, the Cerros del Abrigos boulder field is
almost certainly an inactive rock glacier.
The boulder fields of the Jemez likely first formed as debris
flows or lag deposits. Lag deposits occur in many geological
settings; for example, there are striking lag deposits in the
Plaza Blanca area, where nearby river terrace gravels provide a
source of large clasts.
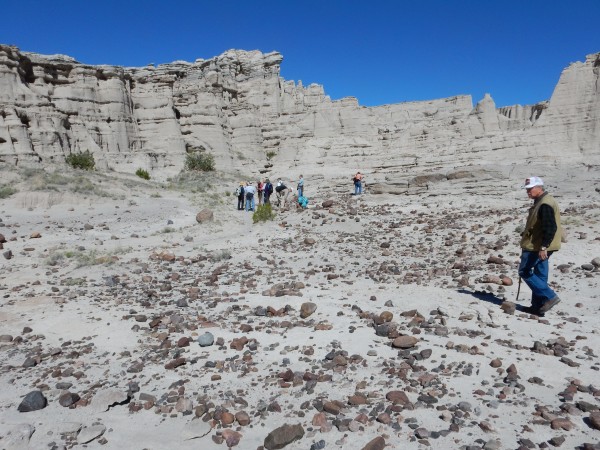
Lag deposits at Plaza Blanca. 36
14.133N 106 18.250W
By way of contrast, there are lag fields on Cerro Rubio and other
locations that show no flow features and were probably never rock
glaciers.
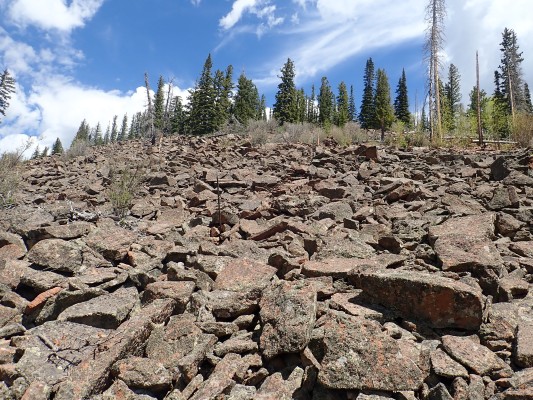
Lag deposits on south face of Cerro Rubio. 35.943137N
106.402056W
The period from 500,000 to 60,000 years ago marked a pause in
volcanic activity in the Jemez. Sometime during this pause, but at
least 200,000 years ago, the first bison arrived in North America.
The appearance in the fossil record of Bison antiquus, the
direct ancestor of the modern bison, defines the beginning of the
Rancholabrean land mammal age of North America, which is named
after the famous tar pits at Rancho
La Brea in what is now Los Angeles. Bison fossil fragments
were discovered just north of the village of White Rock in the
1990s.
Homo sapiens likely also arose during this time period,
perhaps around a quarter of a million years ago. Homo
neanderthalis appeared at about the same time, and the two
closely related species would share the planet until at least
40,000 years ago. Or longer: There are indications that modern
humans carry some Neanderthal genes.
A final burst of
volcanism
The Jemez is not an extinct volcanic field, and will likely erupt
again someday. There are, in fact, indications that a new cycle of
volcanic activity has already commenced — though I hasten to add
that there is little likelihood we will see an eruption in our
lifetime.
The most recent eruptions in the Jemez are those that produced
the El Cajete Pumice, the Battleship Rock Ignimbrite, and the
Banco Bonito Flow, all of which have similar chemistry, seem to
have originated from closely spaced vents, and are likely the
result of a fresh batch of magma rising into the Valles magma
chamber. Collectively they are known as the East Fork Member of
the Valles Rhyolite.
The El Cajete
Pumice
A short distance to the south-east is a most curious crater, an
area of oval form and about one-third of a mile in diameter,
level as a floor and surrounded on two sides by the wall of the
La Jara mountain [Redondo Peak], while the remainder of its
periphery is formed by a low dam of lava. The area is partly
covered by a fine growth of pine timber. The natives call this
crater "el cajete", or the washtub. It is from this region that
the great flows of obsidian and obsidian breccia have evidently
been derived.
-- C.L. Herrick, 1900.
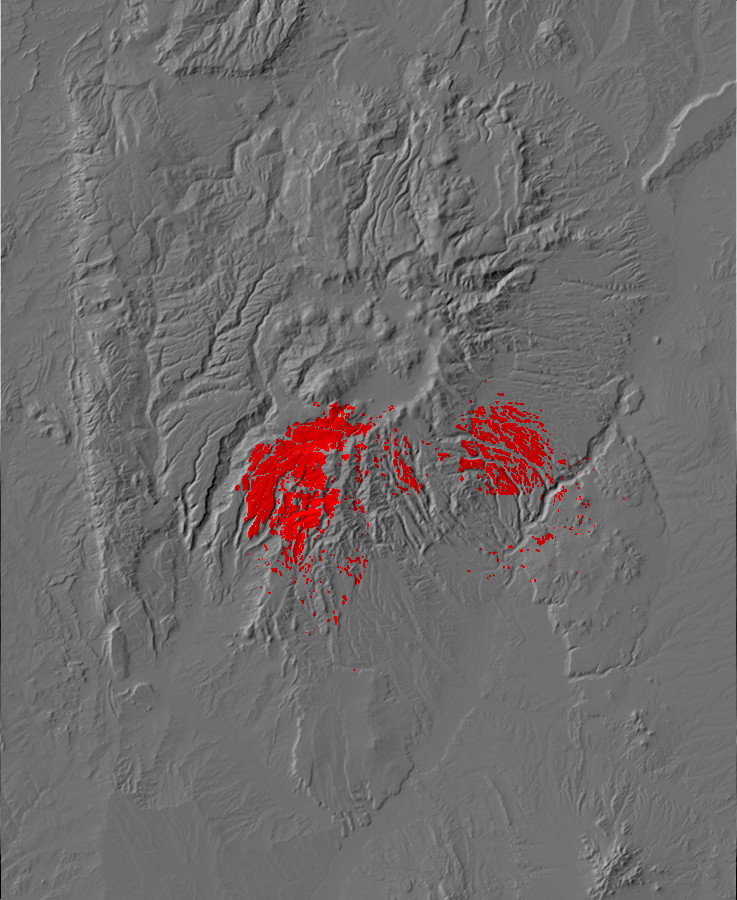
Relief map of the Jemez with El Cajete beds highlighted
in red.
Around 74,000 years ago, a vent opened south of Redondo Peak at El
Cajete. The date of this eruption was long very uncertain.
This is because very young rocks are very difficult to date
precisely by the usual radioisotope methods. There simply isn't
time in a hundred thousand years for enough argon to accumulate
for a reliable radiometric date based on the usual potassium-argon
dating. Charcoal from burnt logs preserved in the volcanic beds
from this eruption shows no measurable 14C, which
means the eruption took place at least 42,000 years ago.
Other dating methods, such as fission track, electron spin
resonance, thermoluminescence, and optically stimulated
thermoluminescence, attempt to measure accumulated radiation
damage to a rock to determine the time since the rock was hot
enough to erase such damage. 21Ne dating is slightly
different in that it measures accumulation of 21Ne in
rocks from cosmic ray bombardment rather than the accumulated
damage from the rock's own radioactivity. These methods yield
highly discordant results, varying from 140,000 years from fission
track dating to as little as 26,000 years from 21Ne
dating. Until quite recently, the accepted estimate, based
mostly on electron spin resonance measurements, was that the
eruption took place around 55,000 years ago.
New high-precision argon-argon dating has helped more precisely
date the El Cajete flows. This technique relies on irradiating the
rock with neutrons in order to convert a nonradioactive isotope of
potassium, 39K, to 39Ar. When done under
carefully controlled conditions and alongside with calibration
samples, this allows the geologist to measure a very precise ratio
of the 39Ar so produced to 40Ar produced
from natural decay of 40K. This in turn yields a very
precise measurement of the ratio 40Ar to total
potassium and thus the age of the rock. High-precision argon-argon
dating of the East Fork Member flows puts their ages very close to
74,000 years.
The eruption produced an eruption column that blanketed much of
the southern Jemez with pumice beds. The map above shows only
those areas where the beds are most substantial. El Cajete pumice
occurs as isolated drifts and thin beds over much of the southern
Jemez and covers many of the Bandelier Tuff mesas in western
Bandelier National Monument.
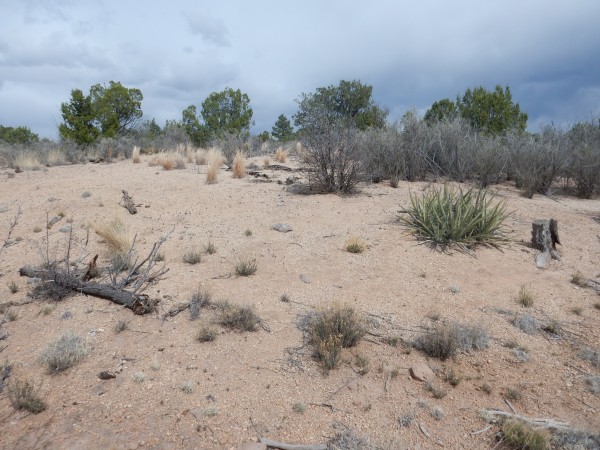
El Cajete Pumice on top of Burnt Mesa in Bandelier
National Monument.. 35
47.419N 106 17.235W
Some beds occur as far east as the Cerros del Rio.
Today, El Cajete is a large meadow south of Redondo Peak.

El Cajete. 35
50.200N 106 33.278W
The flat area is mostly alluvium, carried in by water after the
eruptions from the vent ceased, but there is a patch towards the
center that is an original deposit of El Cajete pumice. There are
also some deep pits in the far side of the flats, used as barbecue
pits for feeding guests when this was part of a private ranch.
At left in the photograph is a rim of pumice thrown up around the
vent. At right is Redondo Peak. The low ridge at center is the
east end of the Banco Bonito obsidian flow, which we'll have more
to say about later on. The vent for the El Cajete pumice is though
to be directly under El Cajete flats, but the vent for the Banco
Bonito flow is further west, not far from the edge of the flow
seen here.
The pumice rim is particularly well-preserved and remarkably
uniform on the southwest side of the vent.
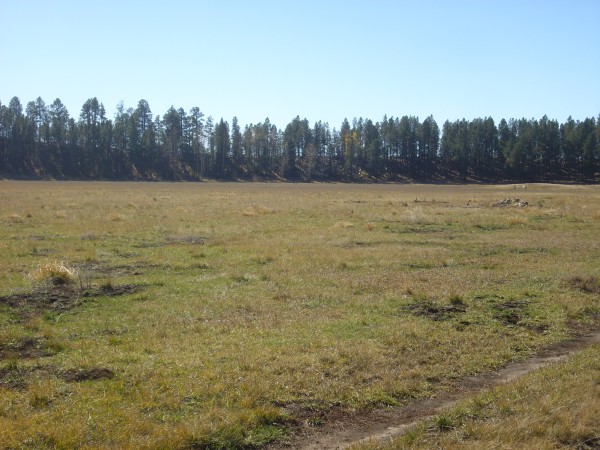
El Cajete. 35.8382376N
106.5652887W
Although the El Cajete eruption produced widespread air fall
pumice beds, the area immediately around the vent has coarser
eruption products. These include large clasts of snowflake
obsidian.
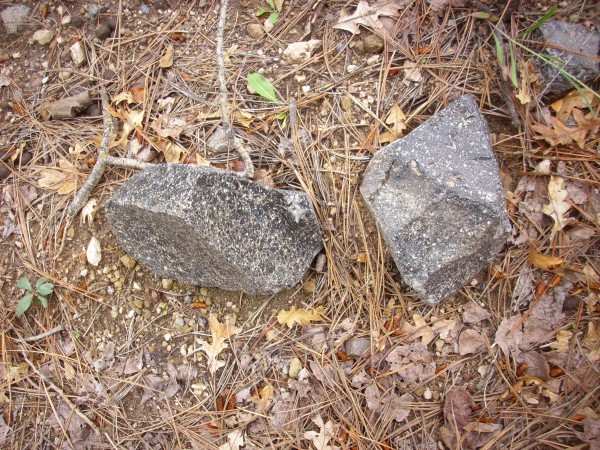
Snowflake obsidian is so named because of the white patches.
These can vary in size from fairly small, as in these samples, to
an inch across.
The white patches are typically cristobalite, a form of
silica that is stable at high temperature. This poses a bit of a
puzzle, as the temperatures measured in modern rhyolite magmas are
not high enough to be in the stability range for cristobalite. The
explanation is given by Ostwald's step rule, which says
that supercooled liquids most easily crystallize to their most
disordered solid form. Cristobalite is less ordered than quartz,
and so a relatively cool rhyolite magma has an easier time
crystallizing to cristobalite than quartz, even though quartz is
the most stable product from very slow cooling.
These clasts are likely remnants of an obsidian dome that formed
on the east side of the vent. Geologists have found evidence for
at least three such glassy domes, each of which marked a pause in
the explosive eruptions of pumice and pyroclastic flows.
Large clasts of vesicular rhyolite are also found close to the
vent.
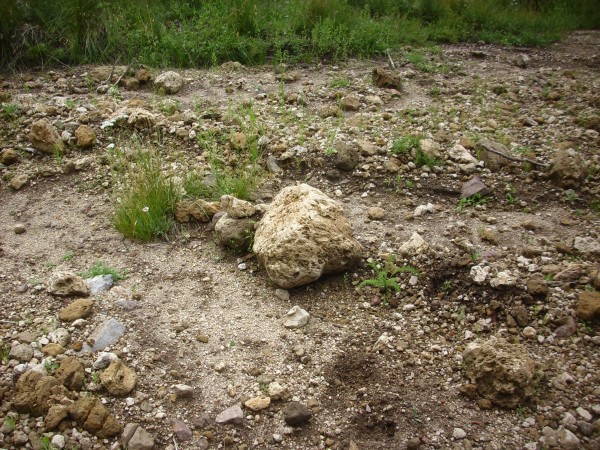
Vesicular rhyolite. 35
50.118N 106 32.746W
Vesicular rhyolite is not bubbly enough to be classified as
pumice, nor massive enough to be just plain rhyolite. The chunks I
hefted felt like tuff. This chunk is larger than a basketball and
is an impressive distance from the vent; given that it’s in a
sheetwash deposit, and given the lay of the land, it probably
originally landed even further from the vent.
Thick beds of El Cajete air fall pumice are exposed in road cuts
along State Road 4 near the source vent.
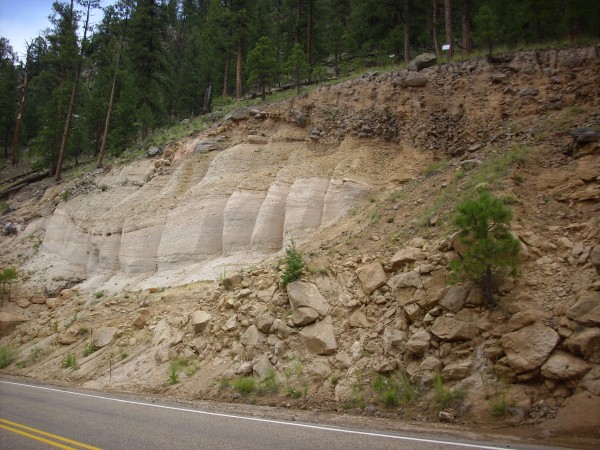
South Mountain Rhyolite, El Cajete Pumice, and Banco
Bonito obsidian in road cut. Near 35
49.675N 106 35.413W
Here the pumice lies on top of South Mountain Rhyolite and in
turn is buried under Banco Bonito obsidian. Here's a sample of the
pumice.
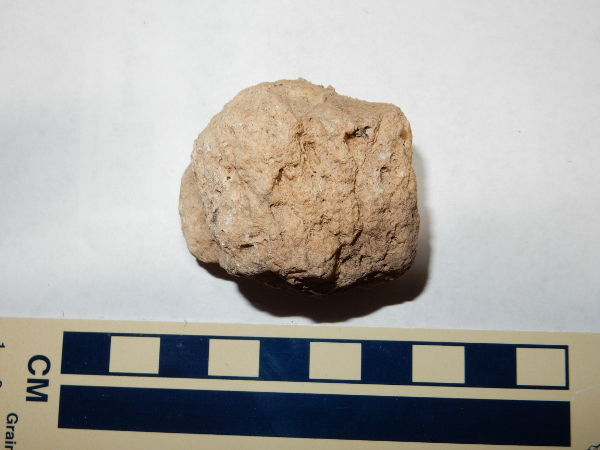
El Cajete Pumice. 35
49.675N 106 35.413W
This sample shows clumps of biotite and hornblende. This is in
contrast to the Bandelier Tuff pumices (Guaje and Tsankawi) which
contain no biotite but have numerous phenocrysts of quartz and
sanidine. Some geologists interpret the mafic minerals as remnants
of the deep crustal rocks from which this magma formed that did not
quite melt.
There is an impressive bed of El Cajete pumice in a driveway cut
as one begins the ascent out of the Vallecitos de los Indios onto
the south caldera rim along Forest Road 10.
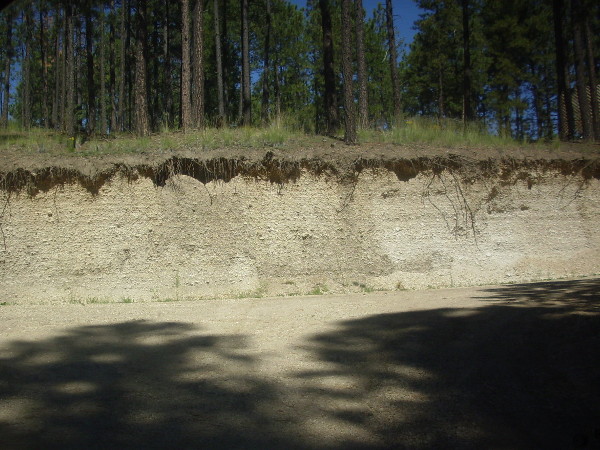
El Cajete pumice. Near 35
48.194N 106 35.400W
El Cajete Pumice beds in the southern Jemez are relatively easily
worked with simple agricultural implements and tend to hold
moisture, and archaeologists have concluded that they played an
important role in Native American settlements in the area. More
recently, they have been extensively mined for use as abrasives,
as an additive in concrete, for water filtration, and as the stone
used to manufacture stone-washed jeans.
Many of the more distant beds are not primary beds (air fall in
place) but have been reworked by streams. Some reworked deposits
are found in Paliza Canyon.
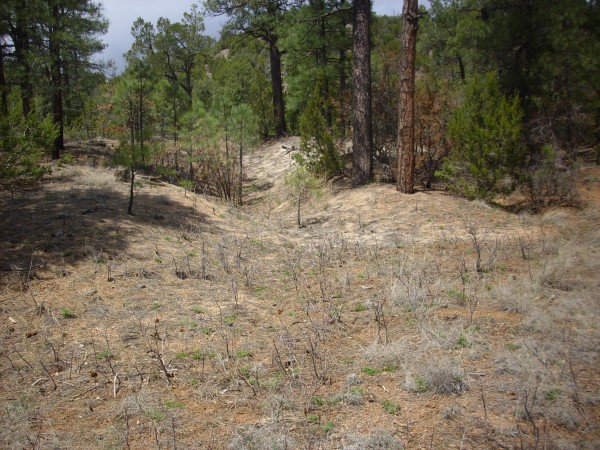
Reworked El Cajete pumice. Near 35
42.763N 106 37.557W
There have been many pumice eruptions in the southern Jemez in
the last eight million years, but El Cajete is a good first guess
whenever loose pumice is found that has no obvious overlying beds.
This can be confirmed by closer examination of the pumice, which
reveals the distinctive mica phenocrysts.
The Battleship
Rock Ignimbrite
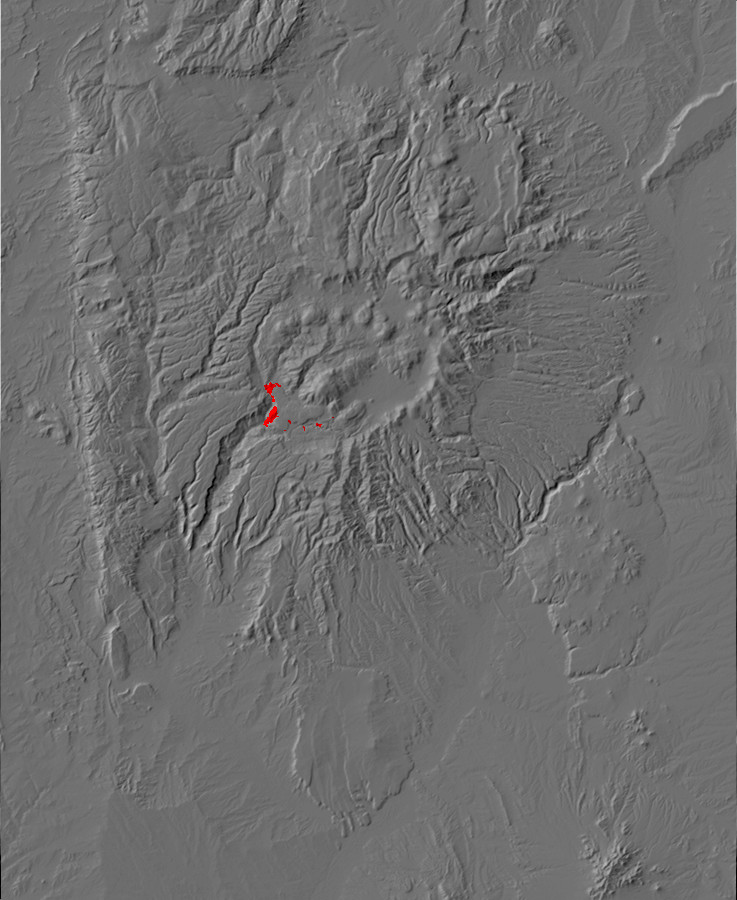
Relief map of the Jemez with Battleship Rock Flow
exposures highlighted in red.
The eruption of the El Cajete Pumice was followed by a series of
pyroclastic flows that generally followed existing river valleys.
These are most prominently exposed at Battleship Rock and along Cañon
de San Diego to the north, where the Jemez River has cut
back through the tuff to reestablish its drainage.
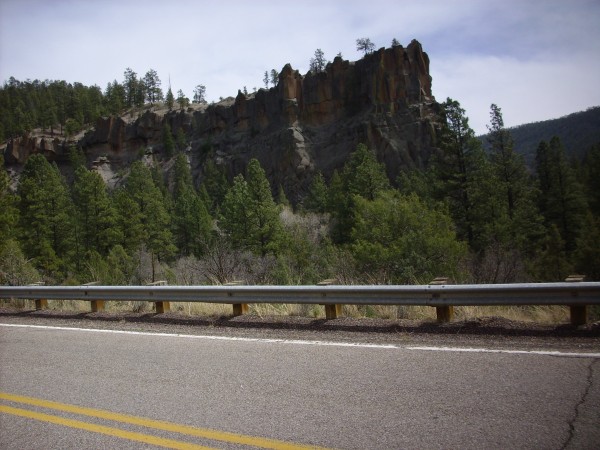
Battleship Rock. 35
49.694N 106 38.551W
Battleship Rock itself marks the confluence of the Jemez and East
Fork Jemez rivers, which have cut through the tuff deposited in
their valleys to leave this dramatic erosional remnant. The rock
is a welded tuff showing classical fiamme:
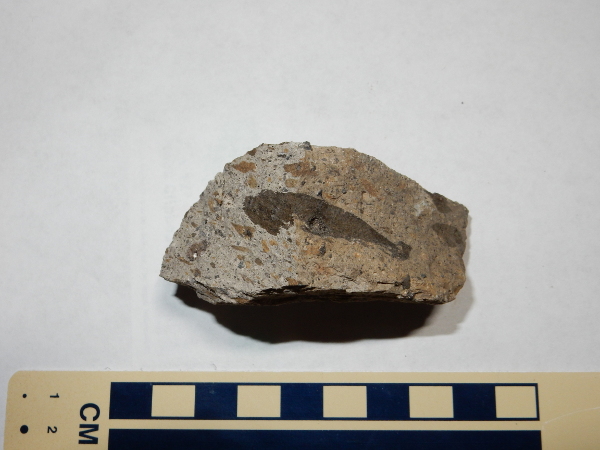
Battleship Rock Ignimbrite. 35
49.694N 106 38.551W
The rock is full of lithic fragments (bits of older rock caught
up in the pyroclastic flow). The elongated brown patches are bits
of soft rock, perhaps pumice or tuff (though the color is rather
dark for that), that were softened and flattened as the very hot
pyroclastic flow settled onto the surface and welded together.
The tuff is quite glassy north of Battleship Rock, so that the
canyon walls glisten under the right lighting conditions.
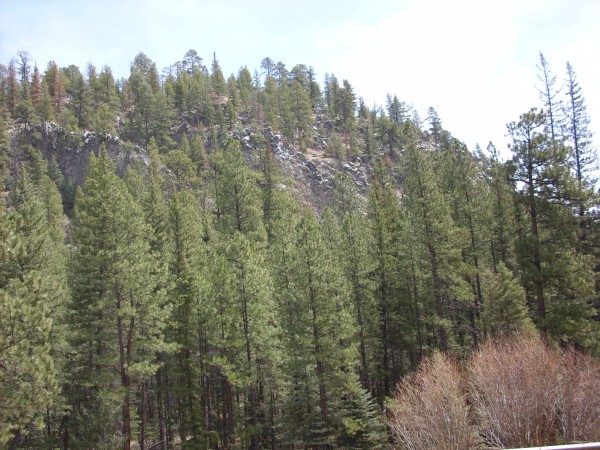
Battleship Rock Ignimbrite. Looking southeast from near
35
50 759N 106 38.075W
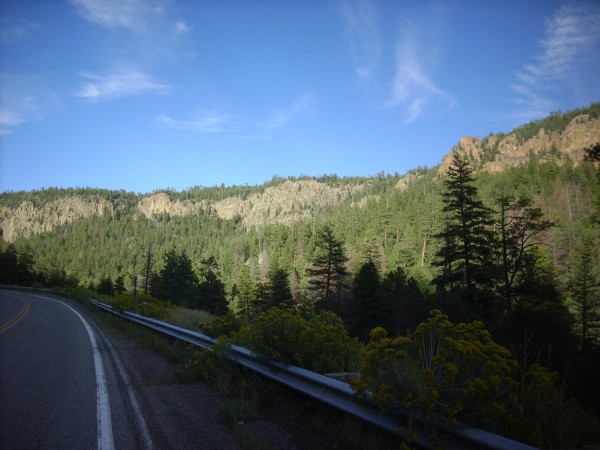
Battleship Rock Ignimbrite at a different time of day.
Looking northeast from 35
50.645N 106 38.185W
Further north, the flow becomes less densely welded and resembles
Bandelier Tuff.
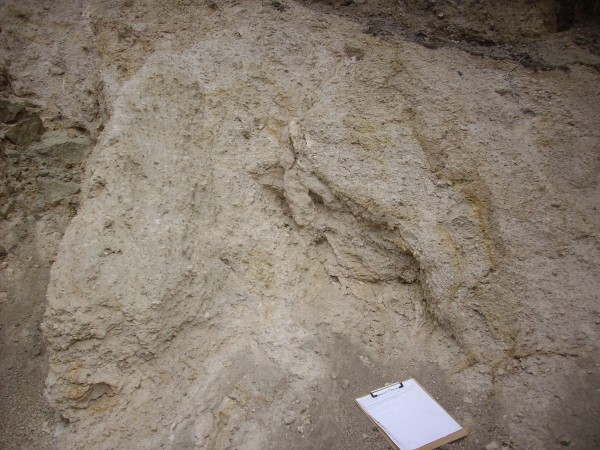
Battleship Rock Ignimbrite at La Cueva. 35
52.289N 106 38.511W
The Banco Bonito
Flow
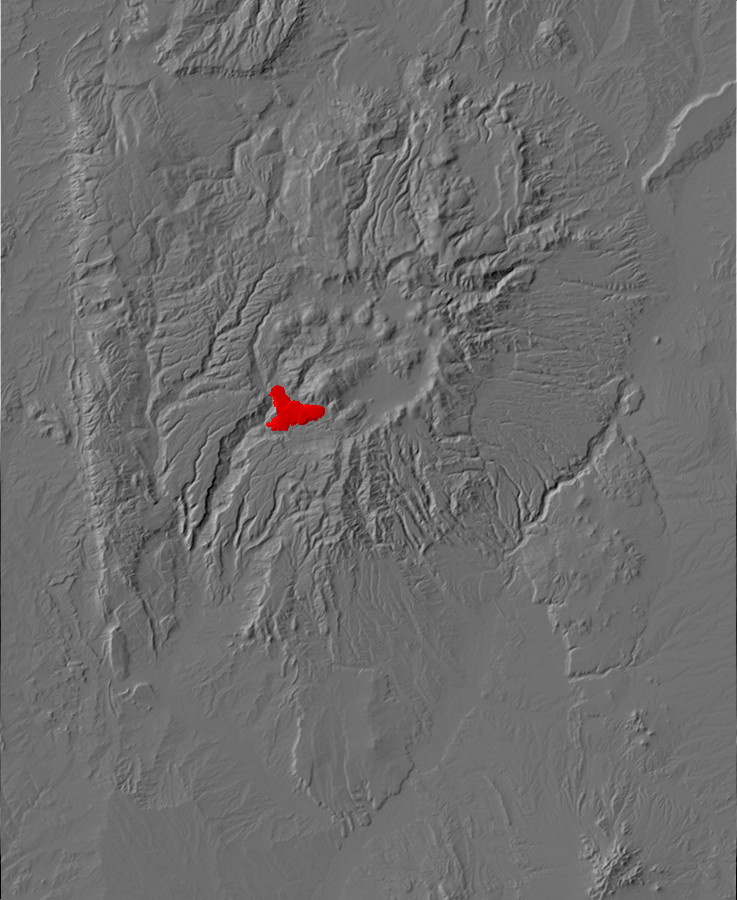
Relief map of the Jemez with Banco Bonito exposures
highlighted in red.
The most recent eruption in the Jemez produced the Banco Bonito
Flow, which fills much of the southwest moat of the Valles
caldera. This flow shows up clearly on satellite
images and relief
maps, because it is young enough to have experienced little
erosion.
Perhaps the best view of the Banco Bonito Flow is had from the
south rim of Valle de los Indios.
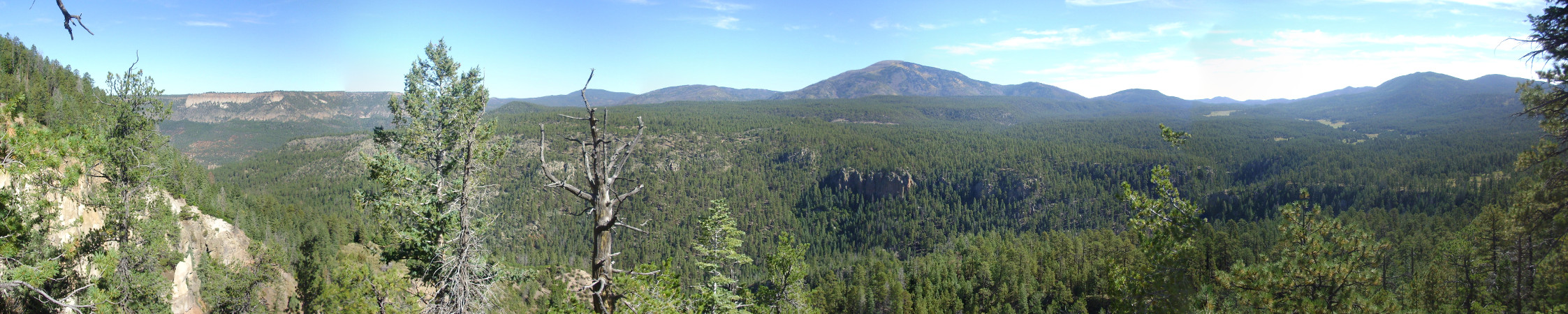
Vallecitos de los Indios from south rim. 35
48.670N 106 37.247W
The panorama begins with the caldera rim immediately to the west,
which is Otowi Formation forming the tent rocks in the foreground.
The distant mesa with prominent cliffs of Tshirege Member, Bandelier
Tuff, is Virgin Mesa on the west side of Cañon de San Diego The red
beds beneath, at the confluence
of Vallecitos de los Indios and Cañon de San Diego, are Permian red
beds of the Abo Formation. The grey outcrop just visible at the
bottom of the canyon at the confluence is Battleship Rock.
At center is Redondo Border and to its right is Redondo Peak.
The knob right of Redondo Peak is South Mountain. Rhyolite of the
South Mountain flows is exposed in the canyon bottom here, beneath
the younger flows of the East Fork Member. The knob to the right
of South Mountain, which is actually beyond Redondo Peak, is Cerro
del Abrigo on the northeast side of the caldera. The two peaks
dominating the right side of the panorama are Los Griegos and
Cerro Pelado on the caldera south rim.
The plateau extending across much of the panorama is the Banco
Bonito flow. This is an obsidian flow, now mostly devitrified. The
rugged cliffs across the canyon are devitrified Banco Bonito
obsidian resting on South Mountain Member flows, with only
occasional small outcrops of the Battleship Rock flow exposed
between the two.
Here's a somewhat closer view, from a stretch of highway where a
recent forest fire exposed the bench.
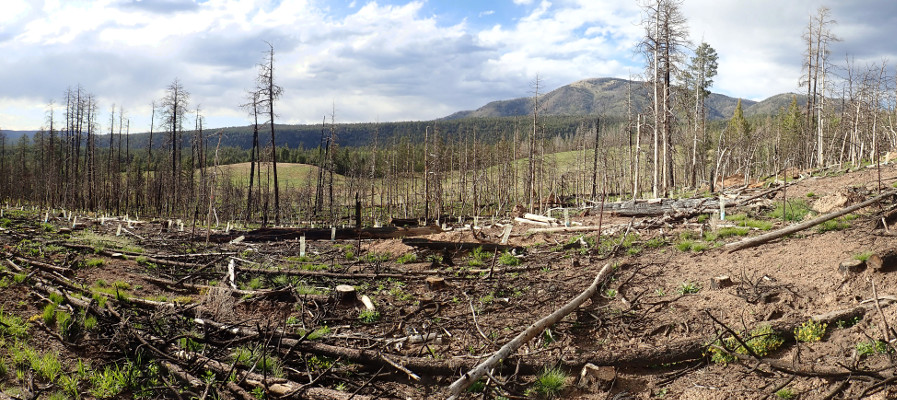
Banco Bonito from Highway 4. 35.8152673N
106.574416W
The forested ridge in the middle distance, extending from and
disappearing behind the trees at right, is Banco Bonito. Redondo
Peak is right of center. El Cajete is located behind the ridge
just to the right of Redondo Peak.
The source vent was likely located just west of El Cajete. Here
the obsidian seems to have formed a dome before flowing into the
lower terrain to the west.
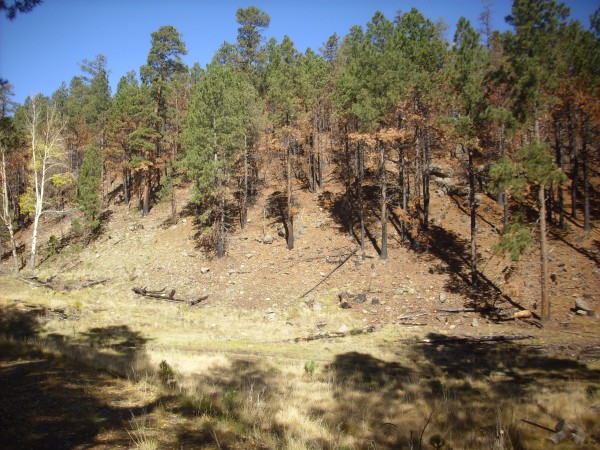
Banco Bonito southeast of the source vent. \35.8358938N
106.5673092W
The slopes here are covered with clasts of partially divitrified,
flow-banded obsidian.
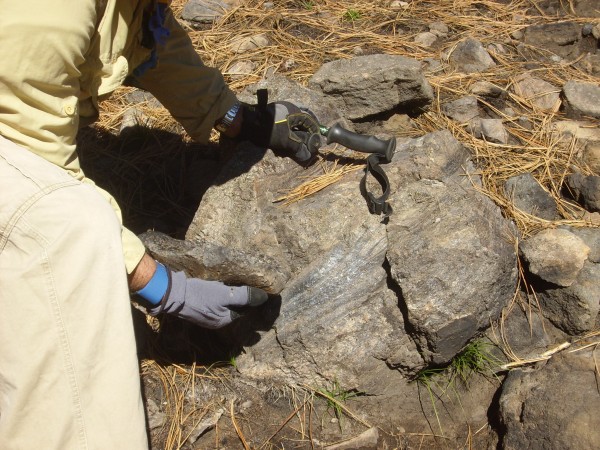
Banco Bonito obsidian near the source vent. Near 35.8358938N
106.5673092W
The area atop the flow near its source vent shows evidence of
pressure ridges.
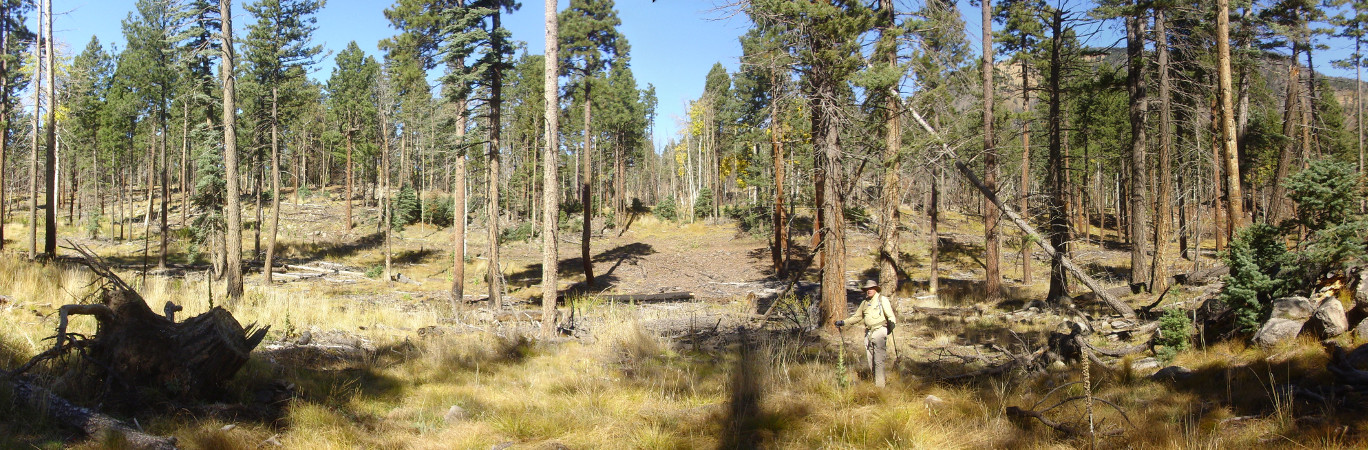
Banco Bonito pressure ridges. Near 35.837752N
106.5682537W
The long hill is likely a pressure ridge, which is a kind of
ripple or wave produced on the semisolid surface of a flow by
movement of still-liquid rock within. There are clearer examples
further west along the Banco Bonito.
Like the El Cajete Pumice, the Banco Bonito Flow is well exposed
on State Road 4 near its source vent.
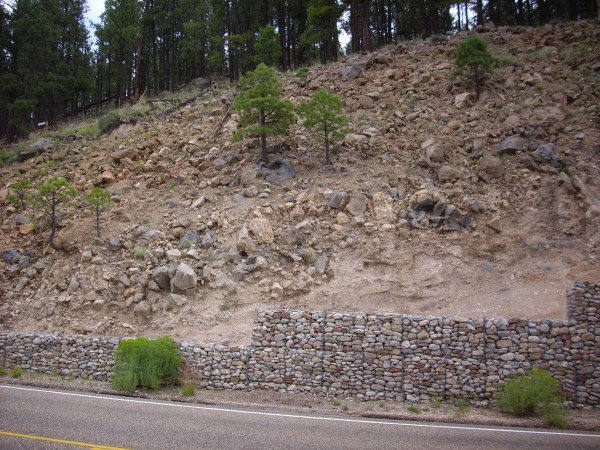
Banco Bonito obsidian in road cut. Near 35
49.675N 106 35.413W
In this area. the road cut has exposed the interior of the flow,
which is only partially devitrified. Much obsidian, though not of
tool quality, is exposed here.
The relief map shows that the flow has a number of large
explosion pits. One of these is located on Forest Service land
just east of State Road 4. Here's a view from the rim of the pit.
Banco Bonito explosion pit seen from rim. Near 35
51.268N 106 37.358W
Here's the view
from the center of the pit itself.

Banco Bonito explosion pit seen from center. Near 35
51.268N 106 37.358W
This kind of explosion pit probably does not represent a source
vent, since these pits are distributed more or less randomly
across the surface of the flow. Similar features are seen at Newberry
Volcano, Oregon, and at other young obsidian flows. The
likely explanation is that they are phreatomagmatic explosion
craters, formed when groundwater accumulates within the upper part
of the flow while its lower portion is still very hot. If drought
lowers the water table, the decrease in pressure can allow the
remaining groundwater to flash into steam and blow out very large
craters like this one.
Support for this theory comes from the presence of broken blocks
of devitrified obsidian in and around the crater.
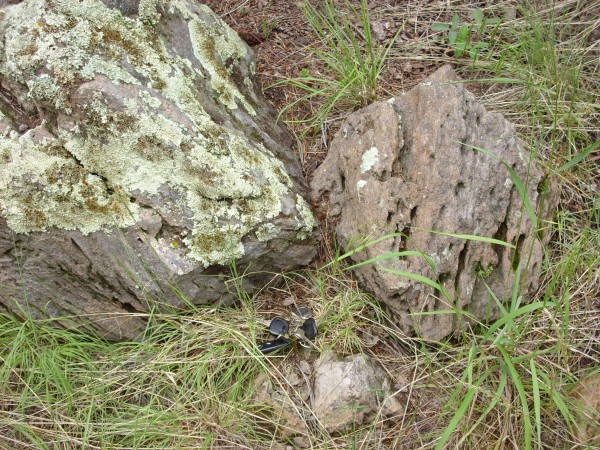
Blocks of Banco Bonito devitrified obsidian. Near 35
51.268N 106 37.358W
Some very young explosion craters of this kind are found at
Yellowstone, and the formation of one such crater in 1989 was
actually witnessed
by geologists nearby.
El Cajete Lake
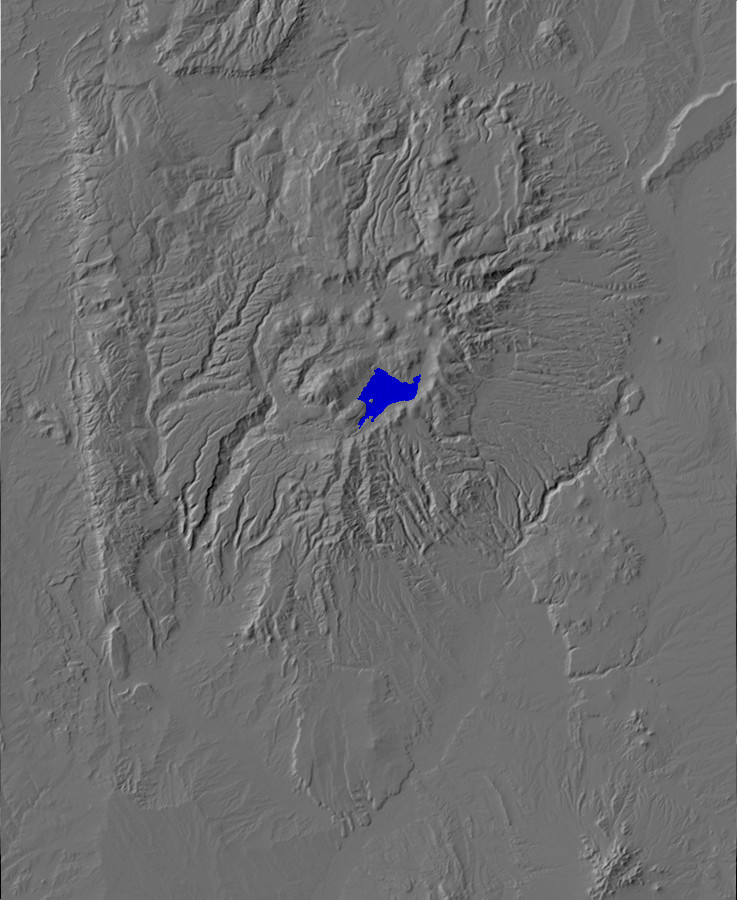
Relief map of the Jemez with El Cajete Lake extent shown
in blue
The fourth known lake in the Valles caldera, following the early
lake and the San Antonio and South Mountain lakes, was the El
Cajete lake. This was formed when the El Cajete and other East
Fork Member eruptions filled the southwest moat and dammed the
East Fork Jemez River. Lake deposits from this most recent lake
are extensive in the Valle Grande, and sometimes contain El Cajete
pumice.
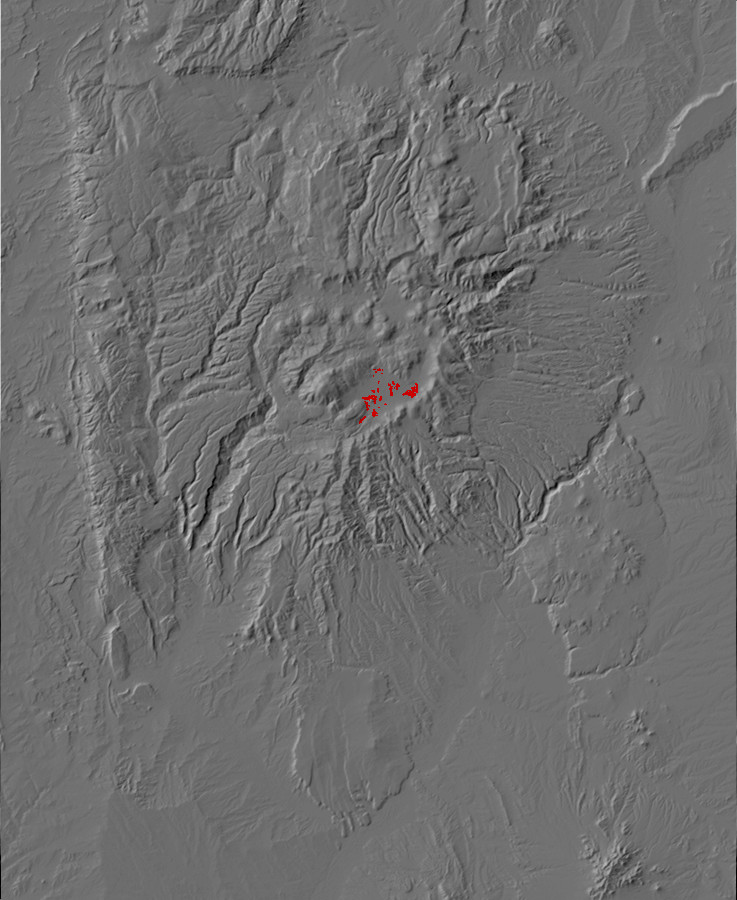
Relief map of the Jemez with El Cajete Lake exposures
highlighted in red.
An exposure of these beds is found southeast of Cerro la Jara.
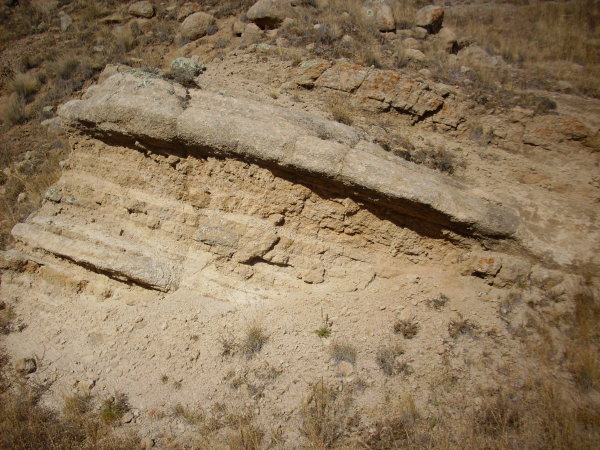
El Cajete lake beds. Near 35
51.155N 106 29.655W
El Cajete Lake also produced lake bars and terraces of loose
gravel. One of these is well exposed in the road cut on the south
flank of Cerro Pinon.
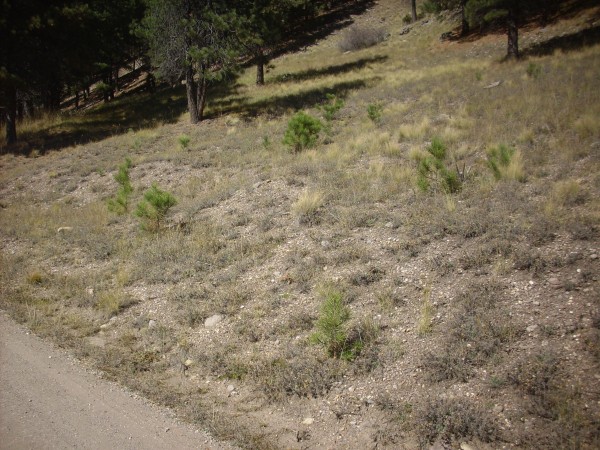
Old lake terrance of El Cajete Lake. 35
53.353N 106 29.714W
The terrace is composed of loose gravel with considerable sand
and clay, containing occasional bits of El Cajete pumice.
Hydrothermal
Activity
In the basin of the sulphurs themselves the walls are chiefly
formed of white tuff and about twenty springs of the most
various character bubble up in the center of mounds of their own
making. Many of these are highly charged with sulphurous oxide
and deposit sulphur in large quantities. Others are highly
carbonated. The deposits of sulphur are of great extent. Though
the accommodations are as yet somewhat primitive and
access very difficult, the result of treatment in the case of
many kinds of disease are often very remarkable.
--C.L.Herrick, 1900, on visiting the Sulphur Springs area.
The Jemez volcanic field is far from dead. It has experienced
volcanism for at least the last fourteen million years, and, as
we've seen, the most recent volcanic activity may have occurred
just 74,000 years ago.
There is evidence that there is still a magma chamber beneath the
Valles caldera. One clue is that seismic waves are seen to slow
down as they pass beneath the caldera. This low-velocity zone
is interpreted as a magma chamber that is only partially
crystallized. Seismic waves passing through the low-velocity zone
also experience higher attenuation than in normal rock.
Another clue is the continuing high rate of heat flow from deep
underground, manifest both in a high temperature gradient in holes
drilled in and around the caldera, and in hydrothermal activity in
the same general area. Hydrothermal activity in the modern Jemez
mostly takes the form of hot springs, though there are a
few fumaroles in the Sulfur Springs area.
The most accessible hydrothermal feature of the Jemez is Soda
Dam. This is a large natural dam of travertine,
calcite deposited by hot water from nearby hot springs.
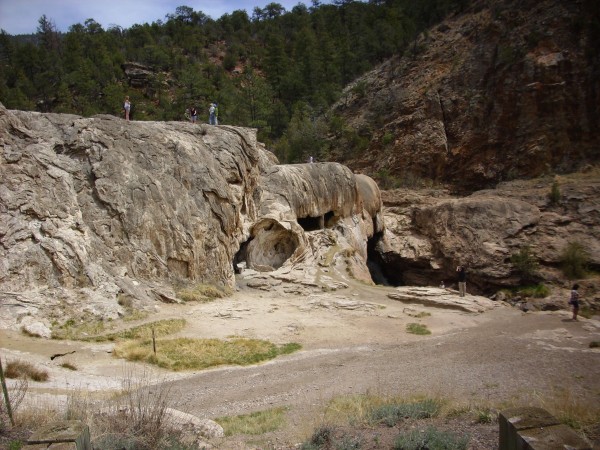
Soda Dam. Looking east from 35.792N
106.687W
There are numerous small hot springs in the area, but the largest
are just across State Road 4 to the west. These originally drained
into a natural plumbing system within the dam. The calcite-rich
water then emerged from a long fracture running the length of the
dam, and the calcite was deposited on the dam surface. Highway
engineers demolished the west end of the dam to improve State Road
4 in 1960 and inadvertently destroyed its plumbing system; the dam
is now slowly eroding away.
Here's one of the feeder springs exposed by the demolition
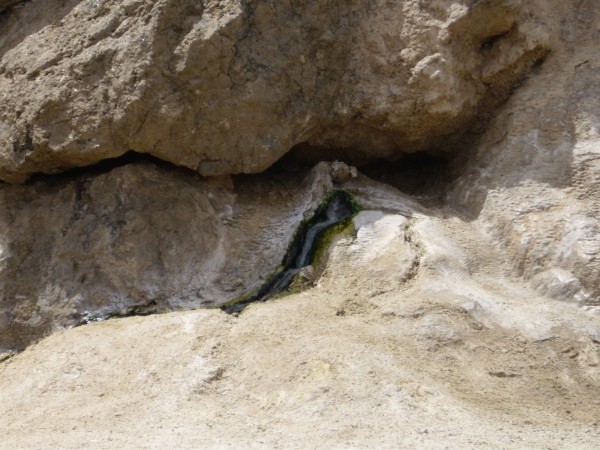
Soda Dam feeder spring. 35.792N
106.687W
The green around the spring flow is partly algae, partly sulfur
bacteria feeding on the sulfur compounds in the spring water. The
smell of sulfur is noticeable throughout this area.
Here's the exposed calcite interior of the dam where it was
partially demolished.
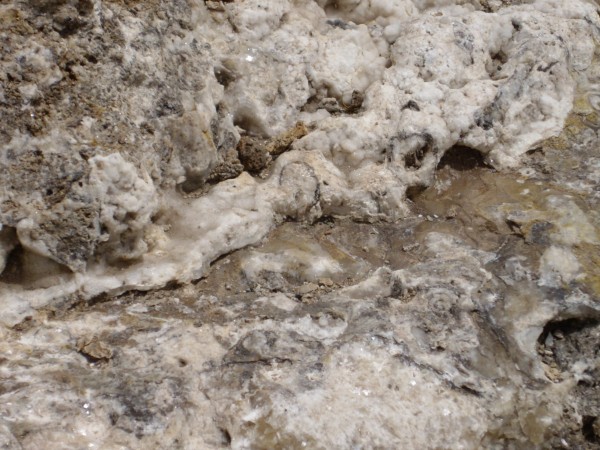
Soda Dam exposed interior. 35.792N
106.687W
The interior is quite coarsely crystalline.
Soda Dam is geologically young, probably less than 5000 years
old. Calcite from the spring waters has cemented together beds of
eroded gneiss from nearby outcrops, which have now been cut by the
road.
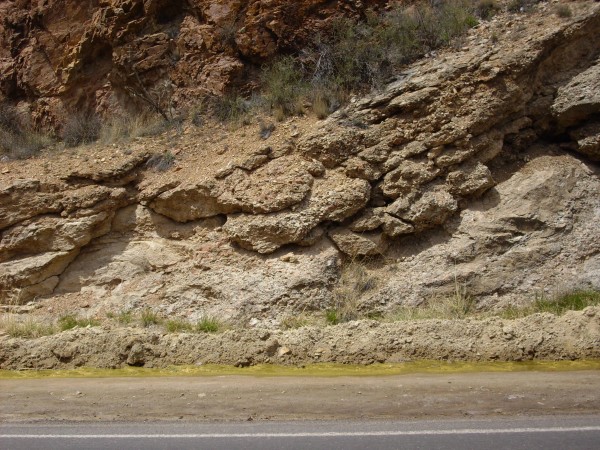
Soda Dam debris beds. Near 35.792N
106.687W
There are a number of older travertine deposits in this area.
Those near river level on the opposite side of the Jemez River are
probably 60 to 110 thousand years old, while those well
above the level of Soda Dam in the nearby canyon walls are around
0.48 to 1.0 million years old. These show that the hydrothermal
system here has been long-lived, and also provide evidence that
Cañon de San Diego was already partially incised within 250,000
years of the Valles event.
The location of the springs is significant. This is the area
where the Jemez Fault crosses Canyon de San Diego, and the fault
zone provides a natural pathway for ground water.
Old travertine is also found along the San Jose Fault, which runs
along the west side of Borrego Mesa.
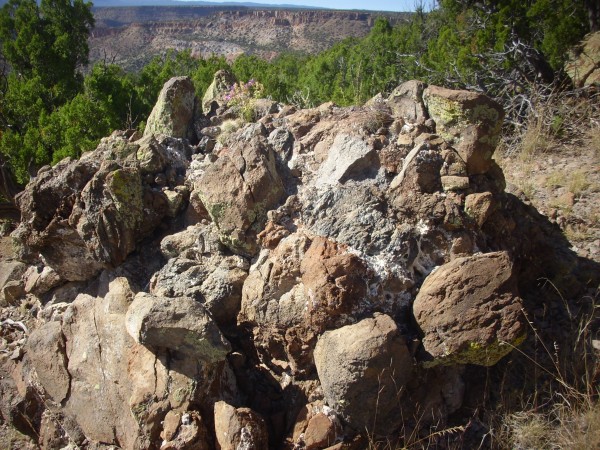
Breccia cemented with travertine. Somewhere near 35
40.618N 106 38.688W
This is one of several stacks of broken rock cemented together
with travertine that are found in this area.
Further down slope there are occasional chunks of travertine in the
landslide debris.
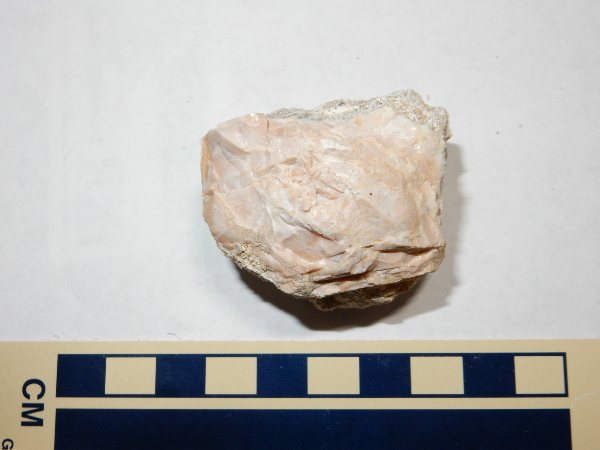
Travertine. Near 35
40.837N 106 38.771W
The identification of this rock as travertine is confirmed both
by its flow features and by the acid test for calcite, which rules
out a flow-banded rhyolite (the only other real possibility.)
The age of the travertine deposits along the face of Borrego Mesa
is unknown. However, stacks like those shown earlier seem to
postdate the landslides, making them less than 70,000 years old.
Not all travertine in the Jemez region is directly connected with
Jemez volcanism. Travertine beds are found near a number of major
faults throughout the area. For example, there are extensive
travertine beds along the Pajarito Fault in the southwest Jemez
and in the Tierra Amarilla anticline to its south. (This is not
the Pajarito Fault Zone near Los Alamos; the duplicate names are
unfortunate.)
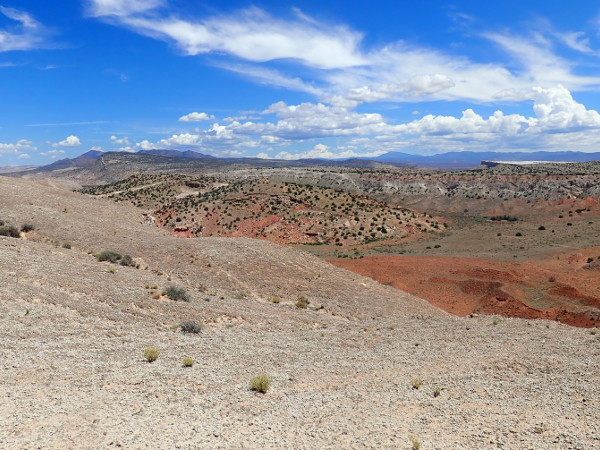
Travertine. Near
35.5089487N
106.8487387W
The hill in the middle of the valley is capped with travertine
deposited by fluids emerging from a nearby major fault.
Springs in the Jemez area fall into three categories. The most
numerous are cold springs, which are recharged by precipitation
that does not penetrate deeply enough to be affected by the deep
magma chamber. These are similar to cold springs found in all but
the most arid climates throughout the world. Their chief
significance in the Jemez is that they tend to follow boundaries
between volcanic flows, where the rock is more permeable, and thus
can serve as clues when mapping flows.
The hottest springs in the Jemez area are found within the Valles
caldera itself, such as at Sulphur
Springs.

Sulfur Springs. 35.908N
106.617W
Sulfur Springs is one of the most geothermally active areas of
the Jemez, and was once mined for sulfur and later developed as a
hot springs resort. Accounts vary on its fate: Some report
that the resort failed during the Great Depression, while others
report that it burned down in the 1960s. Now all that is left are
the surviving buildings of the resort, abandoned trailers, and
other detroitus.
Here the groundwater has been superheated by the very hot rock
beneath the caldera floor, and it is full of sulfur compounds that
give it a very low pH. These acid-sulfate hot springs tend
to rapidly alter rock in their vicinity to clays, iron oxides, and
sinter (amorphous silica.) The area immediately around the
springs is underlain by Deer Canyon Member tuffs, but these have
been altered to light clay minerals by the hydrothermal activity
in the area. The smell of sulfur is unmistakable.
West of the resort is a bank of heavily altered Deer Canyon
Member tuff.
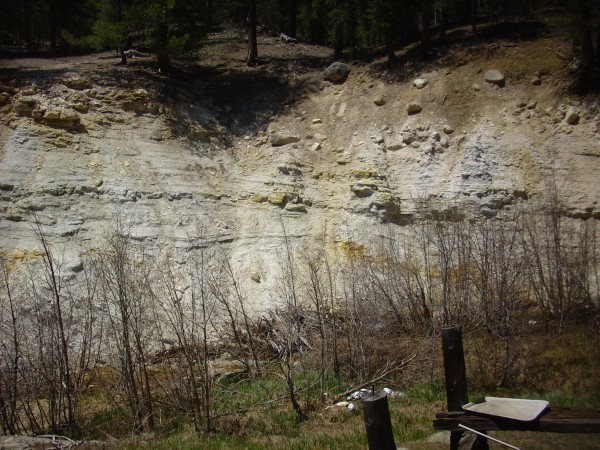
Altered Deer Canyon Member tuff. 35
54.521N 106 36.977W
Another area of warm springs is found at the mouth of Alamo
Canyon. This is a rocky area with bubbles emerging from
several places. In some cases, there was what appeared to be
sulfur deposits around the springs.
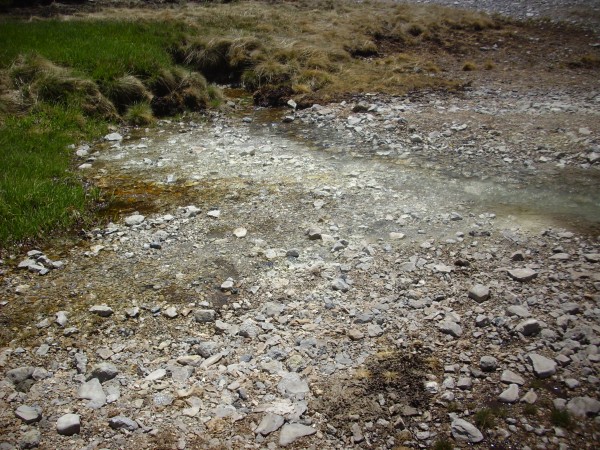
Springs at mouth of Alamo Canyon. 35.919N
106.604W
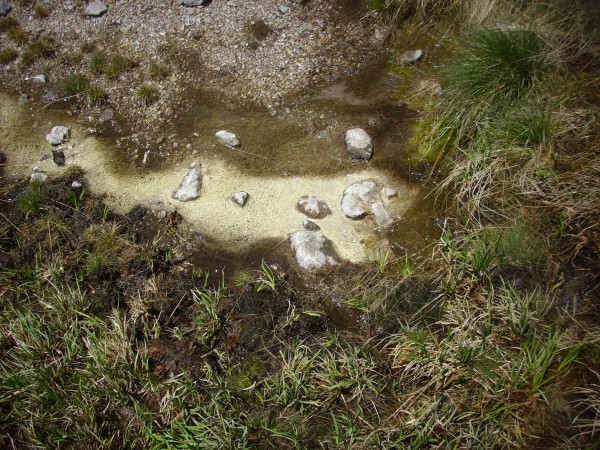
It is hard to imagine that this is anything but sulfur deposited
by the spring. However, at the time I visited the area, the water
was icy cold. I tried several vents; only one was lukewarm rather
than cold. I also found that the water had no noticeable taste. My
visit was in May, and the hot springs may have been diluted by
heavy spring runoff. However, one road log for the area
reports that it is fairly normal for the spring water here to be
cold.
Outside the caldera, one finds warm springs that are dominated by
chloride rather than sulfur compounds and have a neutral to
slightly alkaline pH. The springs in the Jemez Springs area fall
into this category. The water from these springs is meteoric
water (water from rain and snow that has not been long
underground) heated by the hot rock under the surface.
The distinction between chloride and acid-sulfate springs is not
a sharp one, and many springs show both chloride and acid
chemistry. The springs around Soda Dam are moderately
acid-sulfate, but neither as hot nor as low in pH as the springs
at Sulfur Springs.
Sulfur and
volcanoes
Sulfur is a moderately common element in the earth's crust, and
it is particularly associated with volcanic activity.
Sulfur has a complex chemistry compared with elements like
silicon, oxygen, or even iron with its two oxidation states.
Sulfur has many oxidation states, ranging from donating six
electrons in sulfates to accepting two electrons in some sulfides.
Most magmas contain some sulfur, but because sulfur has a strong
affinity for iron, the amount of sulfur in a magma is closely tied
to its iron content. However, sulfur is highly incompatible with
silicates in all but the sulfate state, where it forms rare
sulphosilicate minerals. Because of this incompatibility, the
sulfur in a magma will often separate into a distinct sulfide
phase rich in iron and other chalcophile
(sulfur-compatible) elements, and this phase can produce rich ore
deposits.
One of the surest signs that fresh magma has risen beneath a
volcano is increased emission of sulfur dioxide, SO2.
This has a characteristic sharp smell and dissolves in water to
produce an acid solution of sulfurous acid. When further oxidized
to SO3, as is likely to occur at a slow rate when
exposed to air, it dissolves in water to produce powerful sulfuric
acid. Other gaseous sulfur compounds emitted by volcanoes include
hydrogen sulfide and sulfur vapor. Hydrogen sulfide has a strong
odor of rotten eggs, while sulfur vapor is typically deposited as
solid sulfur crystals near fumaroles.
The most sulfur-rich magmas are alkaline basalt magmas, which can
contain up to 5000 parts per million of sulfur. A value of 1000
parts per million is typical of more common basalts.
Hydrothermal
alteration
We saw earlier that hydrothermal alteration is common in the
lower beds of the Paliza Canyon Formation exposed in canyon
bottoms and the base of the Valles topographic rim. Hydrothermal
alteration is not easy to date, but this episode of alteration
probably immediately followed the eruption of the Bearhead
Rhyolite around 7 million years ago. A second period of
hydrothermal alteration began with the Toledo and Valles eruptions
and continues to the present day.
Here is a rather spectacularly colored rock in an area of the
Valle Jaramillo/Redondo graben saddle mapped as Deer Canyon
Member.
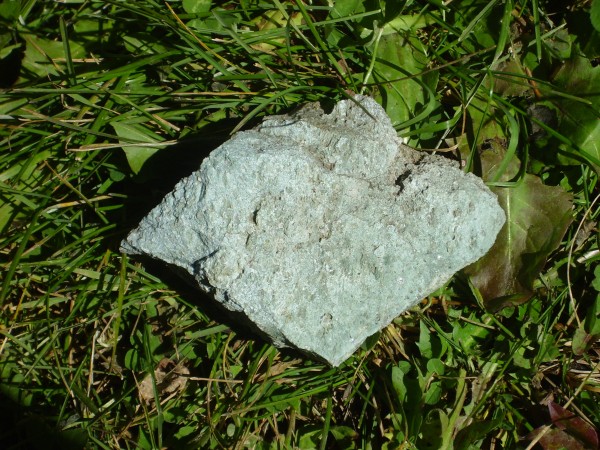
Altered Deer Canyon Member. 35
54.159N 106 33.490W
The vivid green color is due to epidote. The rock is still very
solid, which rules out significant alteration to clay. There are
visible quartz crystals, as well as transparent lath-like crystals
whose identity I’m not mineralogist enough to identify.
Hydrothermal alteration is pervasive in the Deer Canyon and
Redondo Creek Formations.
Erosional
landforms
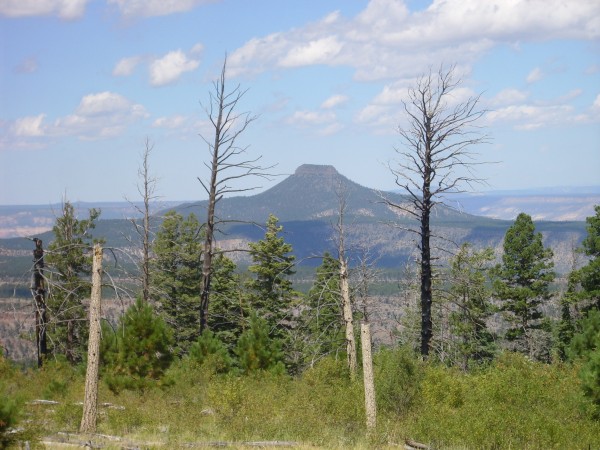
Cerrp Pedernal. Looking west from 36
04.664N 106 25.280W
The landforms we see in the Jemez today are usually eroded,
sometimes heavily. Northern New Mexico has experienced regional
uplift over the last several million years, with the result that
erosion has accelerated and is now destroying rock beds that, in
some cases, are nearly 1.8 billion years old.
It seems kind of sad.
Weathering and
soil
Erosion begins with the weathering of solid rock, which in some
cases produces a layer of soil over the bedrock. Soils show great
variety, even in as small a region as the Jemez Mountains, and I
can only touch on a few aspects of soil formation.
There is now fairly general agreement among geologists that
chemical weathering is the most important process for destroying
solid rock, with mechanical weathering playing a secondary role at
best. Mechanical weathering includes such processes as cracking
from repeated thermal expansion and contraction by day and night
and wedging from water freezing in existing pores and cracks.
Chemical weathering involves slow dissolution of certain mineral
consitutents by rainwater, which is slightly acid, and
groundwater, which varies considerably in pH. Rainwater typically
dissolves sodium, potassium, and calcium out of minerals
containing these elements, such as feldspars and micas. This
converts these minerals to soft clay minerals. For example, the
weathering of potassium feldspar can be represented by the
reaction:
3 KAlSi3O8 +
2 H2CO3 +
12 H2O ⇌ KAl
3Si3O
10(OH)
2 + 6 H4SiO4 +
2 K+ +
2 HCO3−
That is, potassium feldspar exposed to dilute carbonic acid in
rainwater disintegrates into illite, a clay mineral; silica
(written here as silicic acid, H4SiO4); and dissolved potassium bicarbonate.
Potassium ion is highly soluble in water, and almost all the
dissolved potassium eventually reaches the ocean, to be recycled
in subduction zones. Silica is much less soluble, with a maximum
concentration of about 0.02 parts per million in water, and in dry
climates most of it will remain in the soil as quartz. Only in
climates much wetter than New Mexico is the silica leached away to
form nearly pure beds of clay, which in the wettest climates can
undergo further weathering to yield almost pure aluminum
hydroxide. This is the origin of bauxite, the principal aluminum
ore.
Calcium-rich feldspar, common in basalt and andesite, weathers to
smectite by a similar process to produce dissolved calcium ions
and silica. This is the origin of caliche in dry climates,
where the calcium is redeposited as a hard later of calcium
carbonate.
Iron can also be oxidized from ferrous to ferric iron, causing
the ferrous minerals to disintegrate. An example we mentioned
earlier was iddingsite, produced from oxidation of olivine in
basalt lava flows.
Desert soils
The lower elevations around the Jemez are semiarid and produce
characteristic desert soils. These are poor in organic matter and
are alkaline, since the scanty rainfall is not efficient at
leaching weathering products such as sodium, potassium, or
calcium. Calcium, in particular, tends to accumulate in the
subsurface as caliche. We saw examples of this in Chapter
6, where the older basalts of the Lobato Formation are often
encrusted with caliche. Geologists estimate that caliche takes at
least a century to form, which is useful for estimating the age of
young surfaces and for estimating time spans of paleosols in older
sedimentary beds.
Another characteristic of desert soil is desert pavement.
This is well developed at some locations on the rim of White Rock
Canyon.
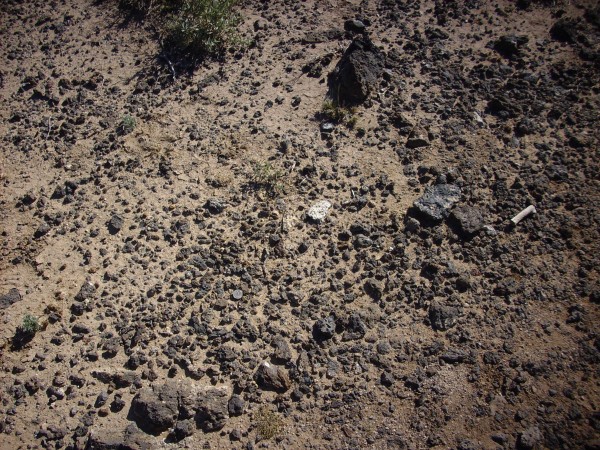
Desert pavement. Note quarter for scale.
Near 35.821N
106.185W
The numerous exposed basalt clasts are an example of a lag
deposit. The remaining soil around the larger clasts is often
cemented, sometimes by hardy cyanobacteria that colonize the soil.
This is fairly delicate terrain, and I'm sorry to say that the
popularity of the area for hiking pretty much guarantees this
desert pavement will eventually be pounded into oblivion. But I
also can't bring myself to tell people to stay away; this is
beauty I can't resist sharing. I'm proud of my home.
Even if there are creepy-crawlies along the trail!
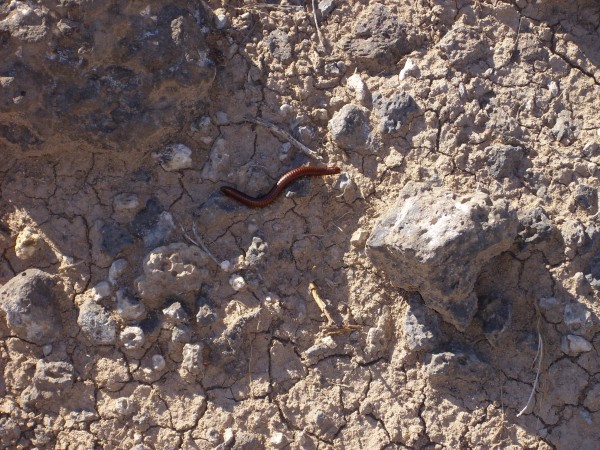
This is Orthoporus
ornatus, the desert millipede. Though uncommon,
tarantulas are also occasionally encountered in the White Rock
area, along with many smaller species of spider.
This reminds me of an experience I had while out walking at
twilight to the local grocery store. It was a pleasant warm
evening, with the light fading fast. I had a head lamp lit so I
could see my way.
I am accustomed to the occasional glint of light from the
ground, from some crystal face oriented just right. But as I
walked along, I saw a pair of greenish glints that rapidly
flashed at me as I walked past. Curious, I moved closer, and saw
that it was two spiders. Their compound eyes had given multiple
green reflections as I moved by.
As I continued my walk, I spotted many more. The spiders seemed
to be mostly of a single species, not large (the biggest had a
leg span of perhaps an inch), brown striped, and clearly some
kind of hunting spider. Of course, I was also a crepuscular
hunter in search of prey, though my prey was an unwary gallon of
milk with which to make my breakfast. Still.
The spiders were beautiful. It was the kind of magic moment I wish
my wife or children had been along for.
Beyond the shadow of the ship,
I watched the water-snakes:
They moved in tracks of shining white
And when they reared, the elfish light
Fell off in hoary flakes.
Within the shadow of the ship
I watched their rich attire:
Blue, glossy green, and velvet black,
Then coiled and swam; and every track
Was a flash of golden fire.
O happy living things! no tongue
Their beauty might declare:
A spring of love gushed from my heart,
And I blessed them unaware:
Sure my kind saint took pity on me,
And I blessed them unaware.
The self-same moment I could pray;
And from my neck so free
The Albatross fell off, and sank
Like lead into the sea.
— Samuel Taylor Coleridge, Rime of the Ancient Mariner
Fire
A significant agent of erosion in the Southwest is wildfire.
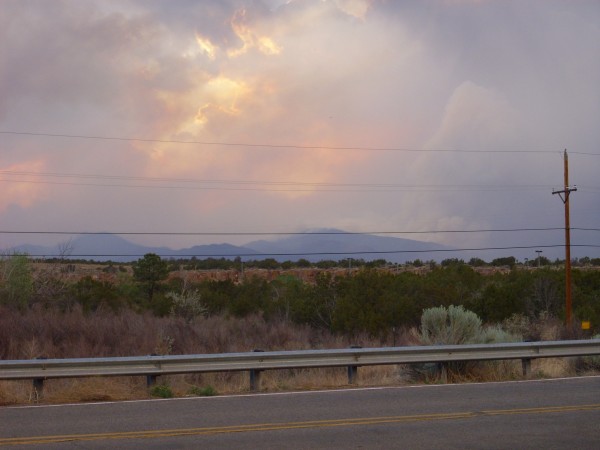
Las Conchas fire of 2011. Looking northwest from
near 35
49.342N 106 13.467W
Under natural conditions, the Jemez Mountains are subject to
frequent, but usually low-intensity, wildfires. Geologists have
taken borings from older trees and stumps in the Jemez and
matched burn scars with tree rings to find the dates of major
fires. It appears that, prior to about 1890, widespread fires
took place every five to fifteen years. These were mostly
surface fires, which burned off grass, shrubs, and tree
seedlings but rarely destroyed more mature trees. The frequency
of fires dropped precipitously in the final years of the 19th
century, probably due to livestock grazing that removed grassy
fuels.
Human efforts since 1900 to reduce the occurrence of fires have
likely had the perverse effect of making fires less frequent,
but more destructive. Without the frequent burning out of ground
debris and younger trees, fuel builds up until devastating crown
fires destroy the forest ecosystem. The trend towards a warmer
and drier climate accentuates the hazard of wildfire. Recent
destructive fires near the Pajarito Plateau include the La Mesa
fire of 1977 (15,444 acres), the 1996 Dome fire (16,516 acres),
the 1998 Oso Complex fire (5,185 acres), the 2000 Cerro Grande
fire (48,000 acres), and the 2011 Las Conchas fire (150,000
acres).
But even before the arrival of humans in New Mexico, wildfires
contributed significantly to erosion. The more intense fires are
capable of speeding the weathering of some kinds of rock.
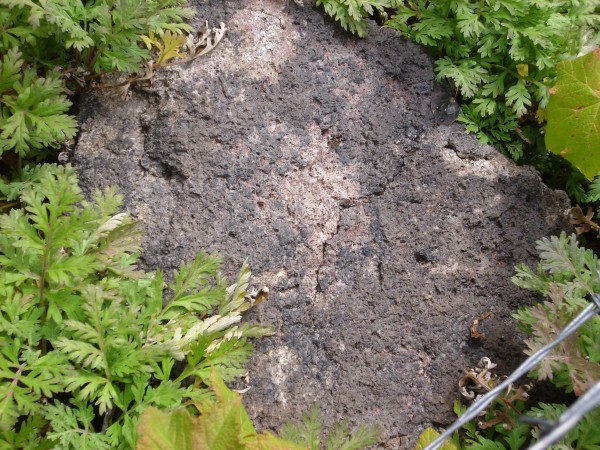
Fire-scorched boulder on Las Conchas.
35
47.838N 106 31.687W
This boulder is in an area that was burned over by the 2011 Las
Conchas fire, a particularly hot and destructive fire, and the
rock show a thin layer of soot. There is also some indication of
exfoliation, the shedding of an outer layer of rock, from
the heat of the fire. It seems likely that this is a significant
cause of weathering over geological time scales in an area where
natural wildfires are a regular occurrence.
Fire also increases erosion by stripping the ground of
vegetative cover. This allows raindrops to hit bare soil and
rainfall to run downhill without the moderating influence of
vegetation. This in turn leads to flash floods, in which
a summer monsoon thunderstorm dropping heavy rain in a brief
period can produce sudden and violent flooding of channels
further down the watershed. These can cause spectacular erosion
in geologically insignificant intervals of time.
Colluvium
Weathering is followed by erosion, in which weathering products
are moved to new locations.
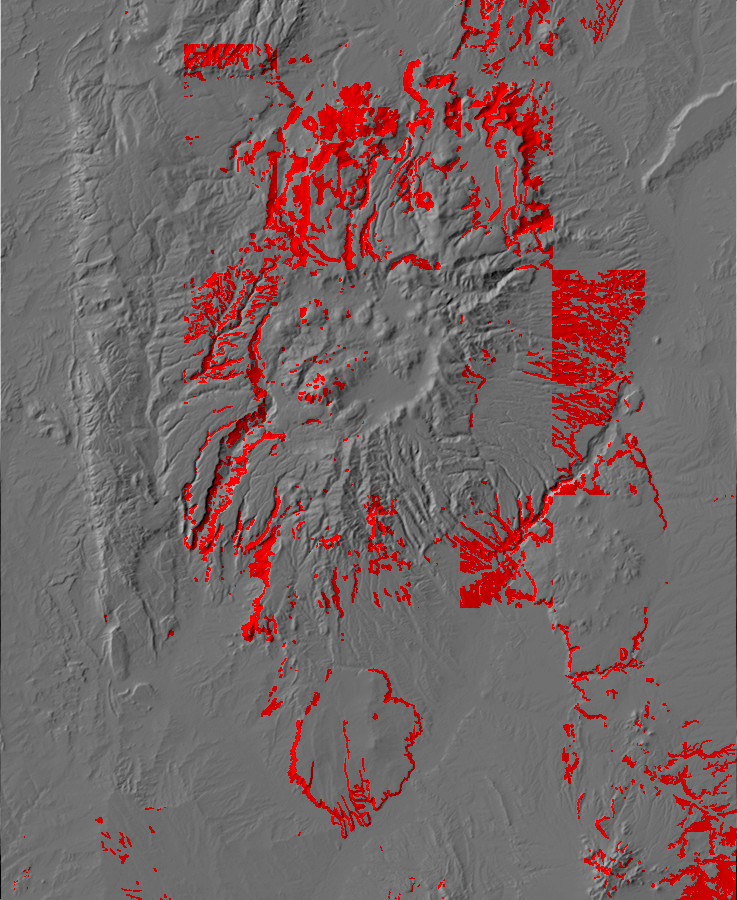
Relief map of the Jemez with colluvium deposits
highlighted in red
The earliest stage of transport of eroded rock in mountainous
areas is the formation of colluvium at the base of
cliffs. In the Jemez area, this is particularly obvious around
mesas of the Tsherige Member, Bandelier Tuff, which typically
are surrounded by talus slopes cut into the underlying
Otowi Member that are covered with clasts of Tsherige Member.
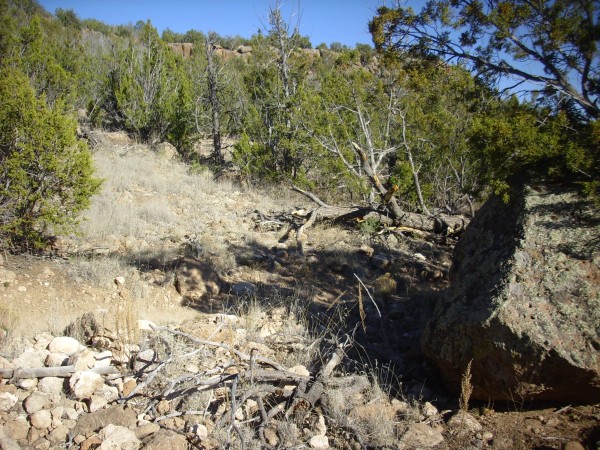
Colluvium at the base of a mesa of Tsherige Member,
Bandelier Tuff. 35.801N
106.224W
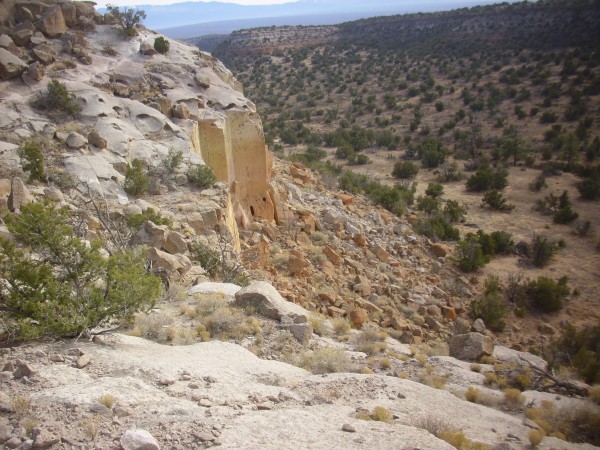
Scree at base of Tsankawi Mesa. 35
48.055N 106 13.433W
Colluvium is unconsolidated fragments of rock and soil that
accumulate at the base of a slope from relatively gentle
processes, such as rainwater flowing over the surface. Colluvium
does not include landslide deposits, which are not a gentle
process, nor alluvial fans produced by the concentrated action
of water. Colluvium is sometimes distinguished from scree,
which is rock shards that have accumulated at the base of a
cliff from which they obviously broken off, but most geologic
maps of the Jemez area have generally mapped it all as
colluvium.
The Otowi Member is often difficult to distinguish from a
colluvium-mantled talus slope at a distance, since it is usually
present only under cliffs of more durable Tshirege Member and it
tends to weather to a gentle slope. For example, as one hikes
down the trail
on the south side of Pueblo Canyon onto the talus slope, one
sees that it is indeed deeply mantled with soil.
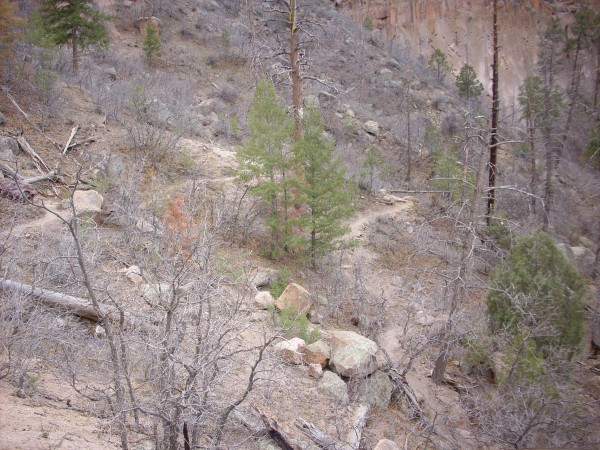
Colluvium on south wall of Pueblo
Canyon. 35
52.890N 106 15.945W
Further complicating the picture is the fact that, because
colluvium is composed of weathered fragments of nearby rocks,
the two can sometimes be hard to tell apart.
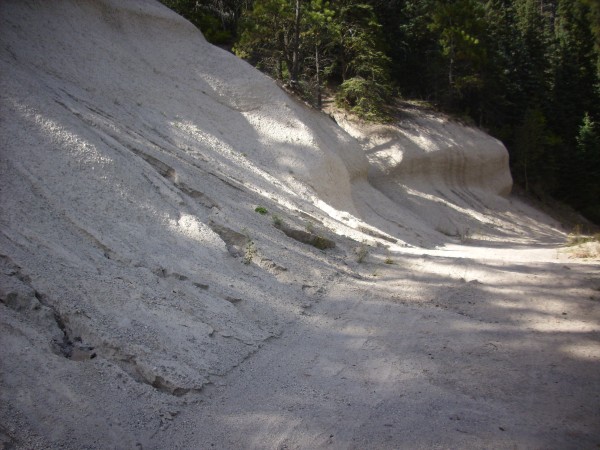
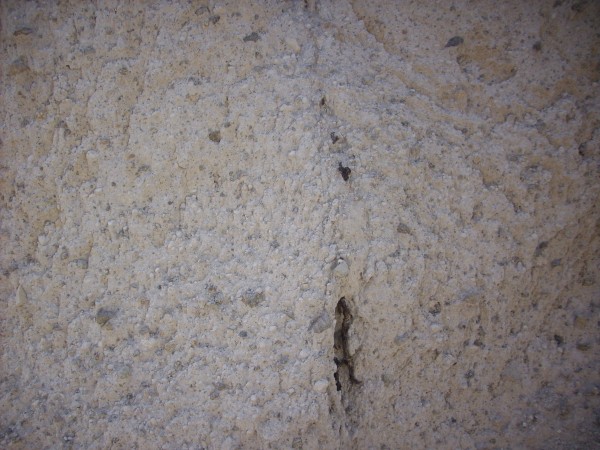
Colluvium formed from Otowi Member in
San Antonio Canyon. 35
56.572N 106 38.791W
The colluvium here looks like a tuff, but it’s very poorly
consolidated. The geologic map maps this area as colluvium, but
shows a bank of Otowi Member just up the hill. So this is reworked
tuff, formed from sediments eroded off the original tuff
bed. It is the historical use of tuff to refer both to
primary pyroclastic deposits and to reworked volcanic ash that
led to adoption of the term ignimbrite to describe
primary pyroclastic deposits. However, the International Union
of Geological Sciences discourages the continued use of this
term, preferring the term ash flow tuff for primary
pyroclastic deposts composed mostly of ash.
Some of the most ambiguous beds are found in the area north of
Los Alamos, where beds mapped as Cerro Toledo sediments in the
Guaje Mountain quadrangle are mapped as colluvium in the
adjoining Puye quadrangle. This accounts for the "boundary
fault" in the map of colluvium deposts east of Los Alamos. The
New Mexico Bureau of Geology & Mineral Resources at Socorro
is presently making a major effort to produce a set of
quadrangle maps for the entire Jemez region that are consistent
across map boundaries.
Landslides
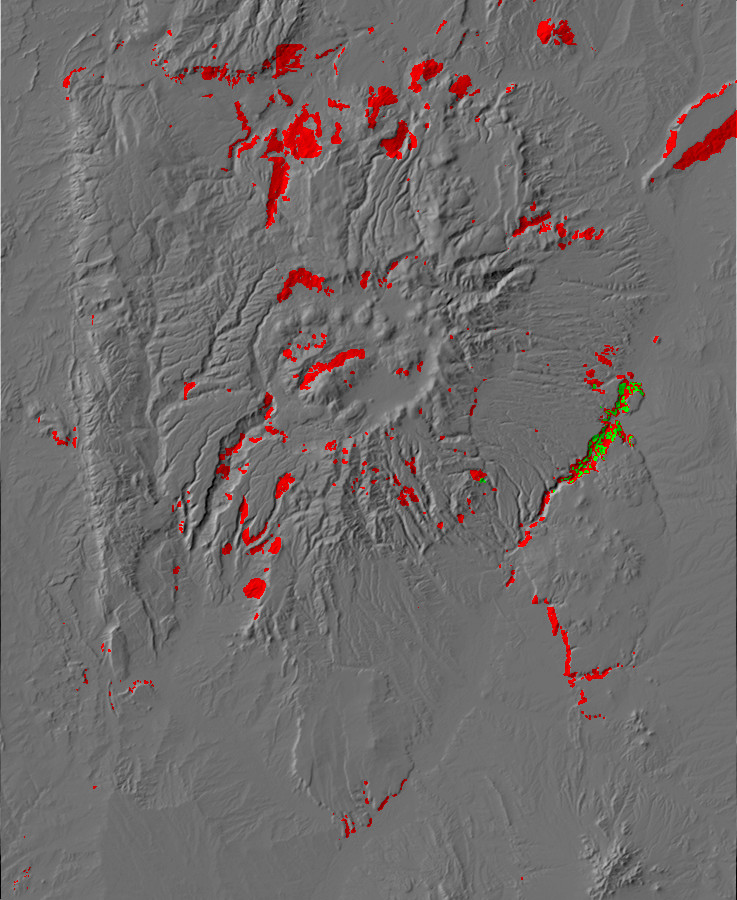
Relief map of the Jemez with toreva blocks of White Rock
Canyon highlighted in green and other landslides
highlighted in red.
Not all rock debris found at the feet of high terrain got there
by gentle processes. The geological picture in many parts of the
Jemez Mountains is of very hard, dense lava flows lying on top of
soft sediments. This is a perfect recipe for landslides.
Toreva blocks in
White Rock Canyon
Some of the most spectacular landslides in the Jemez area are in
White Rock Canyon, where Cerros del Rio basalt overlies poorly
cemented sediments of the Tesuque and Chamita Formations of the
Santa Fe Group. Because the basalt is so durable, the mass wasting
in White Rock Canyon consists of what geologists call Toreva
blocks. These are named after the location they were first
recognized (Toreva,
Arizona.) An entire block of the canyon rim comes loose as a
unit and slides partway down the canyon wall, more or less intact,
so that the original bedding is still present in the block. A
particular characteristic of these blocks is that the block
rotates slightly towards the surface from which it detaches.
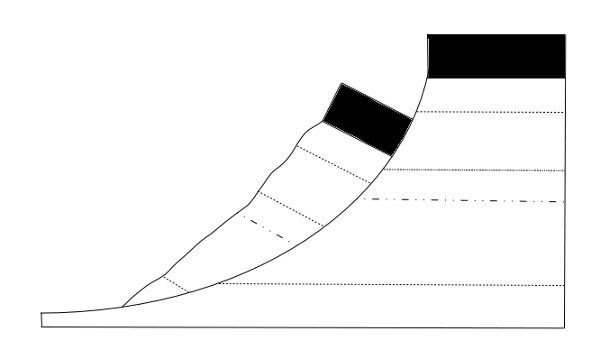
Diagram of Toreva block
The Toreva blocks in White Rock Canyon include some of the best
examples known to geologists.
From the west canyon rim, south of Overlook Park, one has a
beautiful view of one of the most impressive Toreva blocks to be
found below the canyon rim. The Google satellite image doesn't do
it justice; try switching to Map Terrain view, although this also
doesn't quite do it justice. Fortunately, it's highly photogenic.
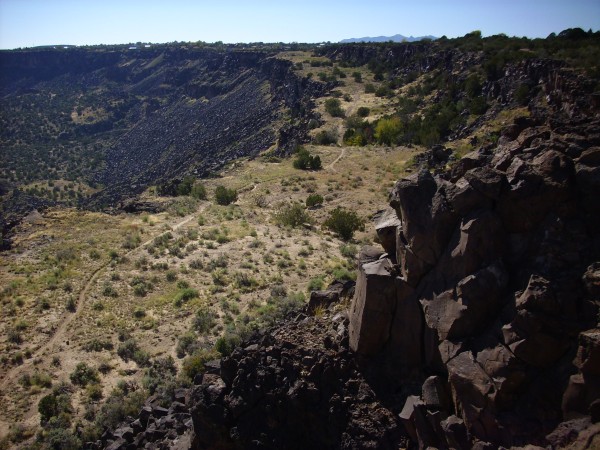
Toreva block on west side of White Rock Canyon Looking
southwest from.
35 48.986N 106 11.422W
The broad meadow is a slice of basalt canyon rim that has broken
loose and slumped partway down the canyon wall. You can see that
the far end of the meadow is nearly at the level of the canyon
rim, and the nearer end has slumped a greater distance, almost as
if the block was hinged at the far end. You can see that there is
a trail down the slump block that affords some nice views of cross
sections of the basalt flows making up the canyon rim.
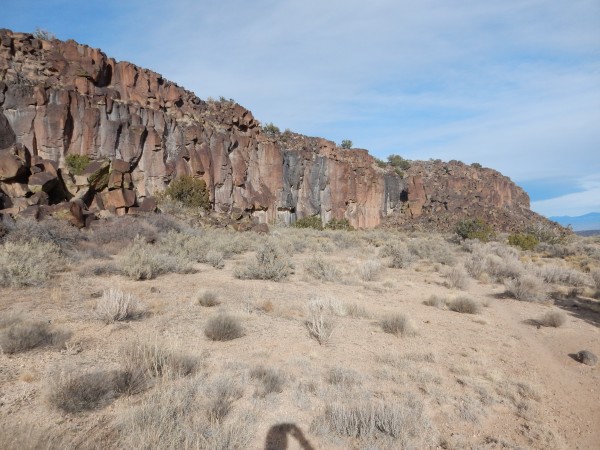
Cliffs on west side of Toreva block.
35 48.948N 106 11.667W
The detachment of the Toreva block has exposed as least two flow
units here. These cliffs are a favorite location for local
rappellers.
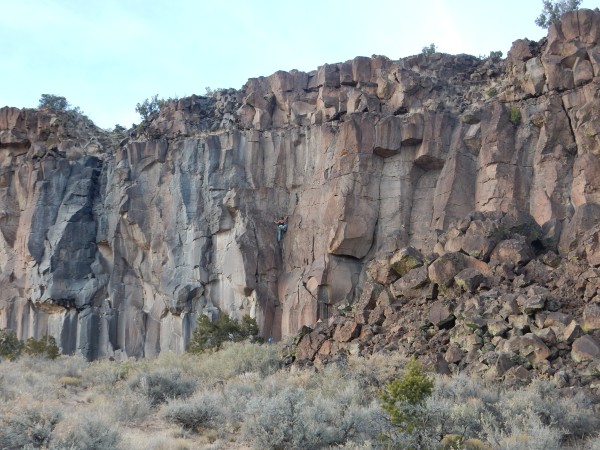
Cliffs on west side of Toreva block with rappeller.
35 48.948N 106 11.667W
The Toreva block gives us a nice view from the canyon rim high
above of hexagonal fracturing of the lava flow. We saw this
picture earlier, in the chapter on the Cerros del Rio.
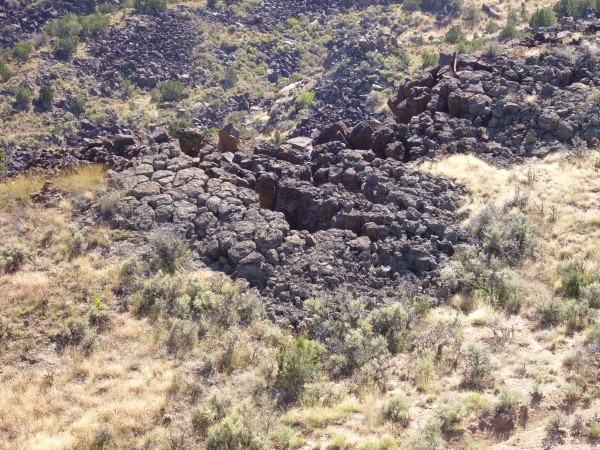
Toreva block on west side of White Rock Canyon Looking
southwest from.
35 48.986N 106 11.422W
Not all coherent detachment produces blocks rotated towards the
cliffs. Relatively shallow slumping of otherwise coherent blocks
can leave the blocks rotated outwards rather than inwards. Some
fine examples are seen just north of the Jemez region, at the
north end of Black Mesa.
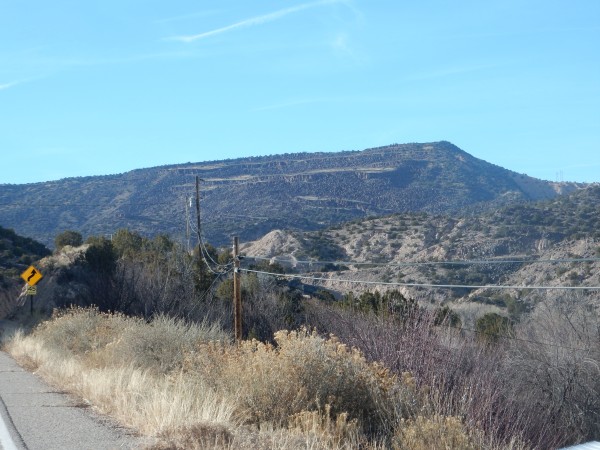
Shallow coherent slumping at north end
of Black Mesa.36
12.727N 105 55.586W
These blocks are rotated slightly outwards rather than
inwards, indicating coherent but shallow slumping that is not of
Toreva type. Such slumping tends not to preserve the lowermost
part of the block as well.
Because of their nature, landslides are difficult to date. Those
in White Rock Canyon are less than 1.6 million years old, judging
from the way that deposits of Guaje Pumice on the rim match
deposits on the Toreva blocks. However, this is only a upper limit
on the age, which is probably much younger.
Borrego Mesa
There are large landslides on the west side of Borrego Mesa. Here
the hard cap is basalt of the Paliza Canyon Formation and the
underlying poorly consolidated sediments are mostly Zia Formation
of the Santa Fe Group. The landslides here do not take the form of
toreva blocks, but more closely resemble a rock avalanche, with
the bedding thoroughly destroyed.
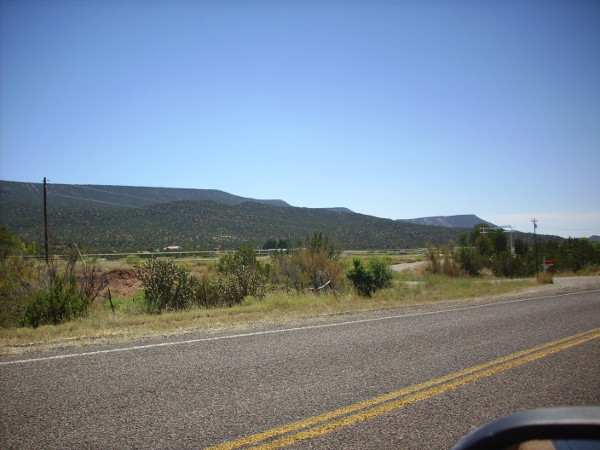
Landslide west of Borrego Mesa. 35
38.671N 106 39.357W
The landslide flows include massive andesite landslide blocks.
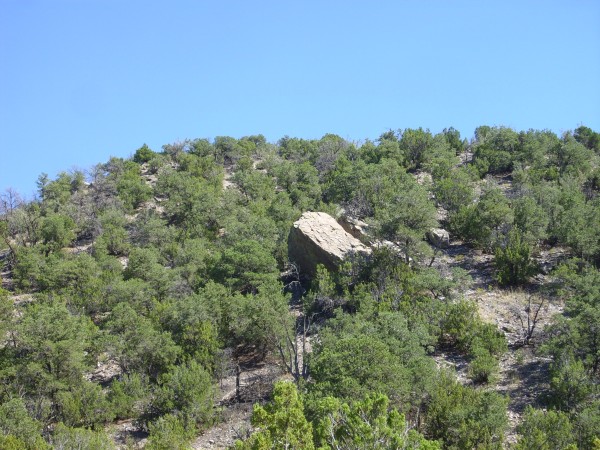
Large landslide block. Near 35
41.063N 106 39.112W
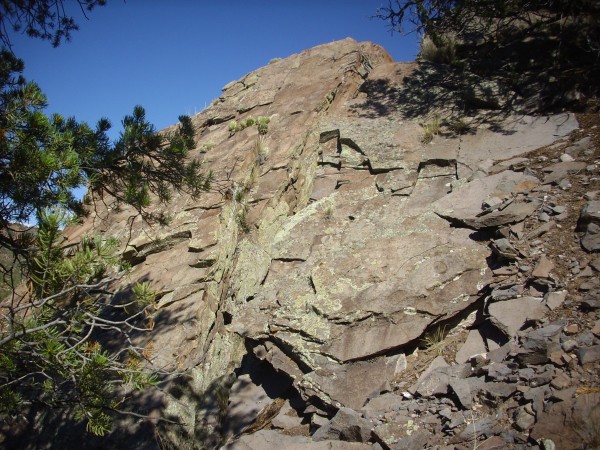
Large landslide block. Near 35
41.063N 106 39.112W
Looking across the valley, one sees smaller but similar
landslides of Bandelier Tuff on Chinle Group sediments.
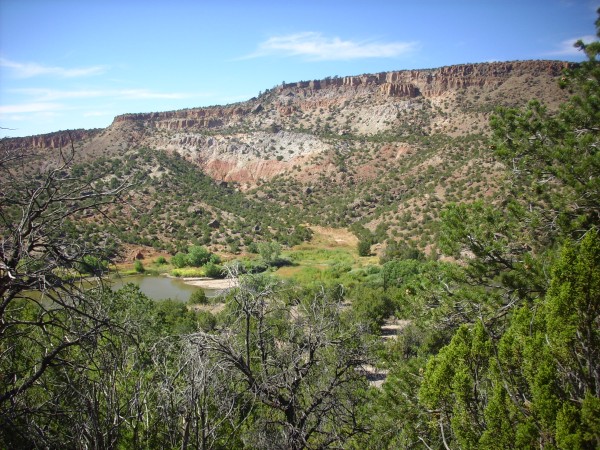
Landslides in Bandelier Tuff. Looking west from near 35
41.063N 106 39.112W
At center, a block of Bandelier Tuff has slumped across the Chinle
Group, which are the red beds underneath. This slump is relatively
coherent and may show slight inwrd rotation typical of a toreva
block.
Most of the landslides appear to be at least 74,000 years old,
the age of the El Cajete Pumice. This is because beds of the
pumice are found in many locations on top of the landslides.
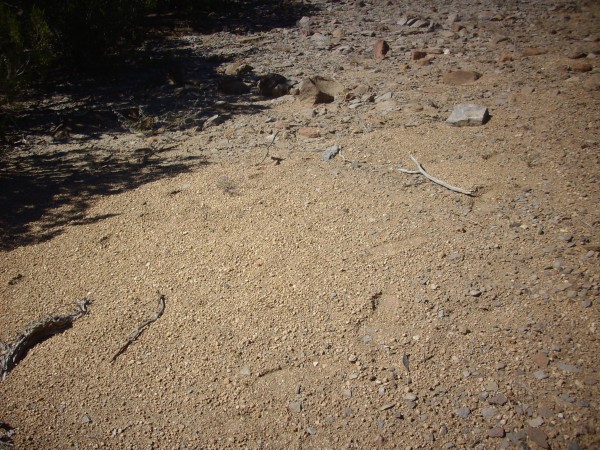
El Cajete Pumice bed on top of landslide. Near 35
40.951N 106 38.709W
Not all the landslides are older than the El Cajete Pumice; a few
small slides in this area appear to lie on top of beds of the El
Cajete Pumice.
The landslide deposits on Borrego Mesa include extensive boulder
fields.
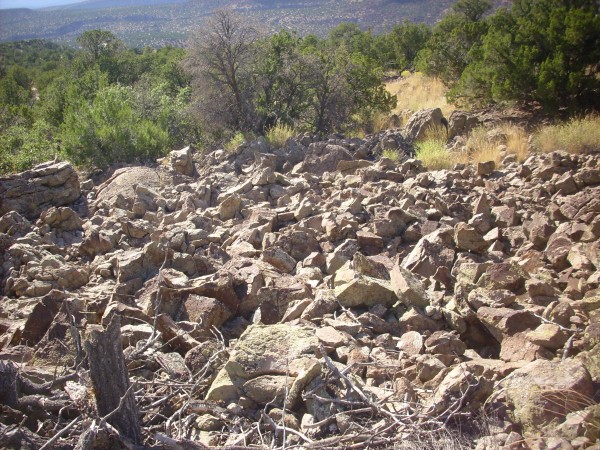
Boulder field. Near 35
40.837N 106 38.771W
This is a rockslide deposit below a prominent knob of andesite,
of the same composition as the boulders here.
San Miguel
Mountains
The northern flank of the St. Peter's Dome volcanic center
collapsed sometime between its emplacement 8.7 million years ago
and the eruption of the El Cajete Pumice 73,000 years ago, based
on the presence of El Cajete deposits on top of the landslide.
Here the landslide blocks were composed of andesitic lavas atop
volcaniclastics that were more strongly cemented than the Santa Fe
Group beds elsewhere. One block survived in more or less coherent
form.
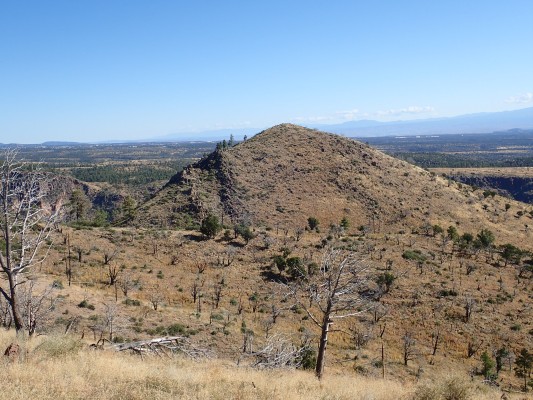
St. Peter's Dome landslide block. Looking north from 35.762888N
106.352715,191W
This is interpreted as a landslide block that was so highly
rotated that the lava beds are left are now oriented vertically.
As with other landslides of the Jemez region, time control is
poor. However, field relationships in Capulin Canyon, at the base
of the landslide, hint that this landslide postdates the Valles
event, and so took place between 1.25 million and 73,000 years
ago.
Vallecitos de los
Indios
The northern slopes of Vallecitos de los Indios are covered with
landslide deposits formed from Banco Bonito obsidian flows atop
mudstone of the Abo Formation. These are relatively accessible,
being crossed by the hiking trail to McCauley Springs. The size of
some of the clasts in the landslide is impressive.
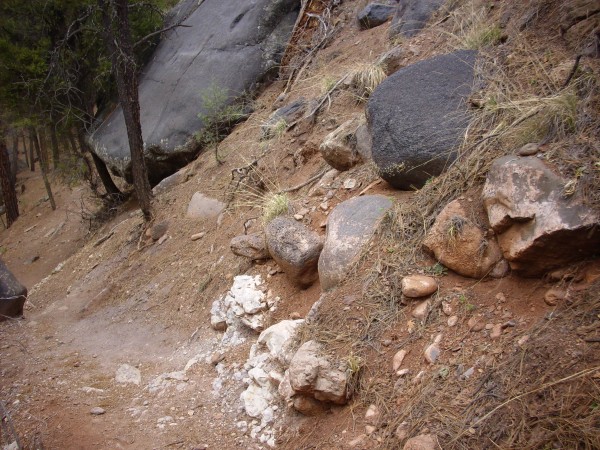
Landslide deposits along the McCauley Springs trail.
Somewhere near 35.824N
106.638W
The largest bounders here are devitrified obsidian boulders of
the Banco Bonito flow. The reddish soil shows its origins in the
mudstones of the Abo Formation.
Alluvial fans
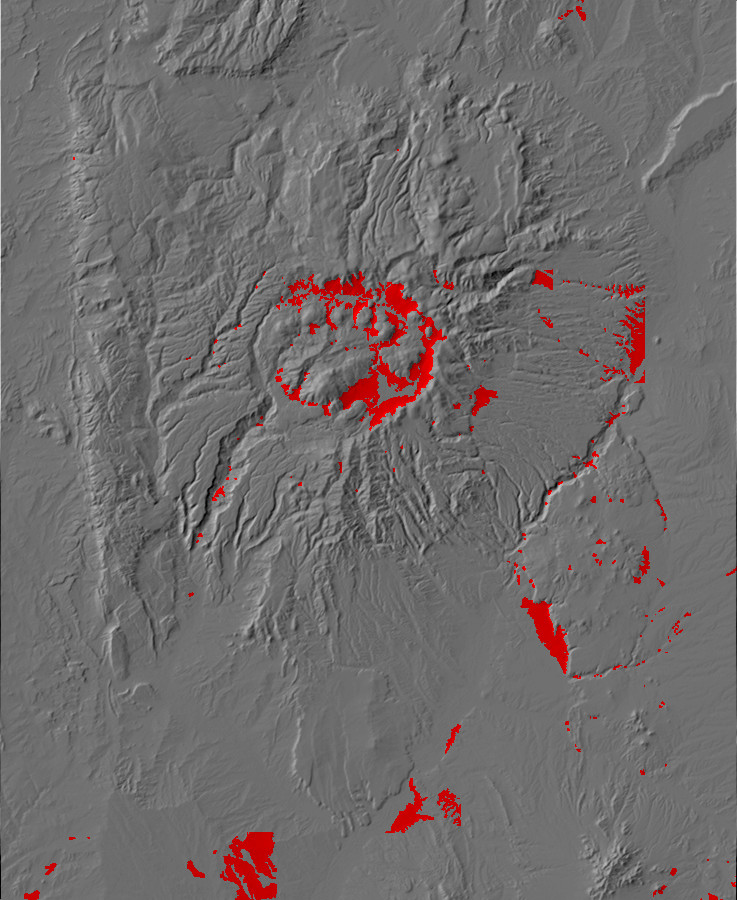
Relief map of the Jemez with alluvial fans highlighted
in red.
Over time, colluvium is washed further downhill by streams and
floods to produce alluvial fans. These are found along
much of the inner rim of the Valles caldera, as well as other
locations of high relief.
The ideal alluvial fan has a distinctive shallow cone shape, with
its tip at a canyon emerging from an escarpment. Here periodic
floods in the canyon emerge, spread out, and deposit their
sediments on the surface of the fan. Alluvial fans can be found in
almost any climate, but are most recognizable in arid climates,
and there are some spectacular alluvial fans in Death Valley and
other parts of the Basin and Range.
Surprisingly, tthe Jemez region lacks well-developed alluvial
fans, even at lower and drier elevations along escarpments. This
may be yet another effect of regional uplift, increasing erosion
rates to the point where fans do not accumulate. More
typical of this area are bajadas, which are coalesced
alluvial fans along an escarpment. These lack the distinctive cone
shape, resembling more of an apron of sediment around the high
ground. They are distinct from pediment surfaces in that they are
composed of deposits of sediments rather than being eroded out of
bedrock. They differ from colluvium in that concentrated flow, not
rainwash or gravity, is the main mechanism of transport.
One can examine an alluvial fan on the Valles
Caldera Trail, part of the Valles National Preserve. The
trail descends the inner rim of the caldera on its southeast to
the caldera floor, where the alluvial fan includes local debris
flow deposits.
Alluvial fan boulder deposits within
Valles Caldera. 35
51.408N 106 26.110W
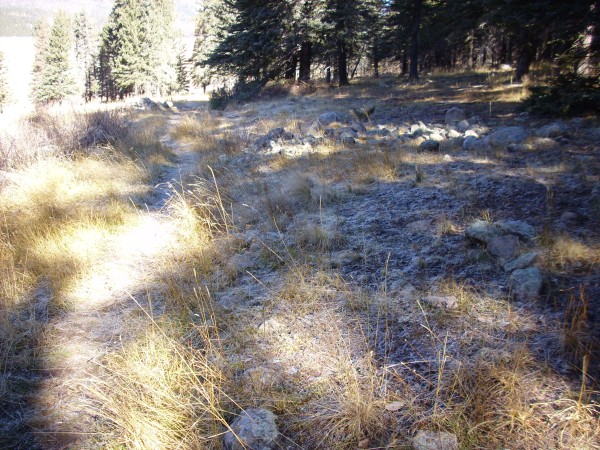
Alluvial fan deposits within Valles
Caldera. 35
51.432N 106 26.169W
Individual boulders are composed of the Tschicoma
Formation dacite of the caldera rim.
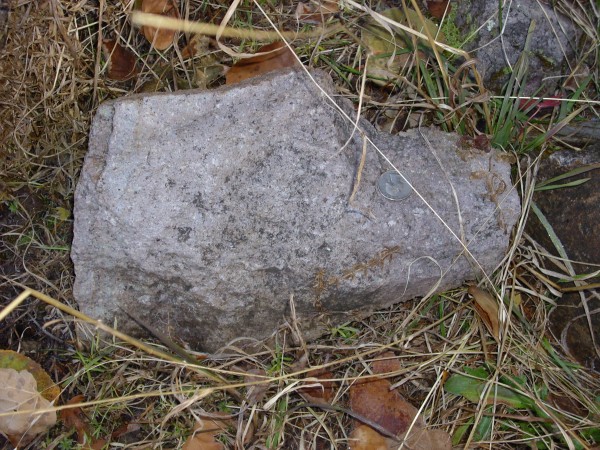
Tshicoma Dacite in landslide on Valles Caldera Trail
Debris flows continue to be a part of the modern Jemez landscape.
They are particularly active in areas that have suffered recent
fire damage, such as the area south of Redondo Peak (which was
burned during the 2011 Las Conchas fire).
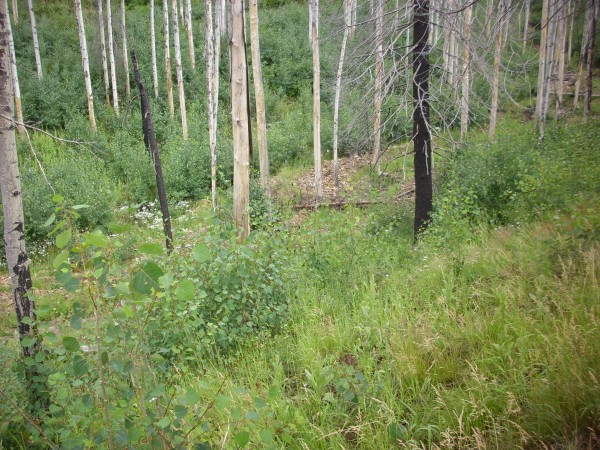
Recent debris flow in drainage near El Cajete trail, south
of Redondo Peak. 35.84N 106.542W
A river runs
through it
The tufa is penetrated at various places by lava flows of
recent basalt. Especially is this true of the region at the
north mouth of White Rock Canyon, where it would appear that the
river that at one time made its way through the Santa Fe marls,
along the edge of the tufa, was blocked by the basalt and set
back to form a great estuary or lake. It may be that at the time
immediately following the period of basalt eruption the
existence of such dams was so general as to have an appreciable
effect on the climate. At any rate there are several instances
of such lava dams.
— C.L.Herrick, 1900
Herrick erred in thinking the Bandelier Tuff was older than the
Cerros del Rio Basalt, but he was right in his observation that
flows of the Cerros del Rio Basalt repeatedly blocked the Rio
Grande in White Rock Canyon.
The presence of the Totavi Lentil shows that the Rio Grande
already existed 5 million years ago, when the Puye Formation began
to be laid down. The history of this river thus overlaps most of
the recent history of the Jemez volcanic field itself. Rather than
trying to "interbed" that story with the story of the Jemez
Mountains, I'm going to step back to 5 million years ago and tell
that story now as a coherent narrative of its own.
The location of the Lentil, well west of the modern Rio Grande
and at a higher elevation, suggests that the Rio Grande originally
ran further to the west and was slowly forced eastward by
accumulating sediments of the Puye Formation. The river was at
least 225m (738') above its current level when it first appears in
the geologic record. The river was not yet fully integrated along
its entire modern valley. The full integration of the Rio Chama
and Rio Grande through the Espanola Basin is thought to have
occurred sometime between 2.8 and 4.0 million years ago.
Once it had eroded through the basalt cap, the Rio Grande in
White Rock Canyon rapidly cut deeply into the underlying soft
sediments of the Santa Fe Group, The river reached its maximum
depth 2.8 million years ago, at a level about 30 m (100') below
the modern level. It left behind a series of terraces to the west,
which are now deeply buried under the Pajarito Plateau and are
known only from test wells. These divide Santa Fe sediments
beneath from Puye Formation sediments above. The maximum depth is
recorded by a flow of olivine basalt very close to the modern
river level near Ancho
Canyon, which has a radiometric age of 2.47 million years.
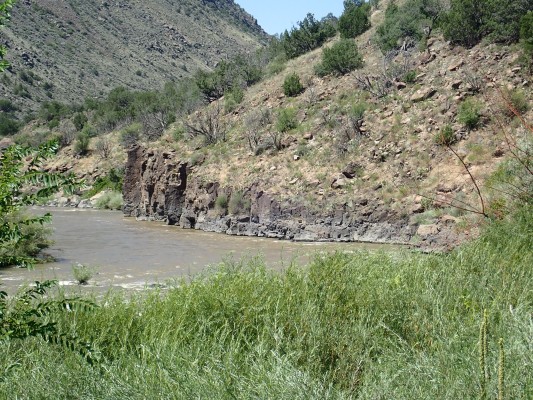
Cerros del Rio basalt flow at river level. 35.770275N
106.223119W
The relatively young age of this flow shows that it must have
followed a deep canyon in the surrounding, much older, Santa Fe
Group beds and early Cerros del Rio beds. This flow marked the
beginning of a series of natural dams, formed by volcanic
activity, that forced the river level back up in upper White Rock
Canyon.
The highest of these dams was a flow of tholeiitic lava at the
current location of Water
Canyon that erupted around 2.46 million years ago. This
drove the level of the river back up to 285m (935') above its
current level. The resulting lake, Culebra Lake, covered much of
the Espanola Basin. It is now believed that much of the
preservation of the Puye Formation can be attributed to Culebra
Lake, which raised the base level for erosion of the Puye
sediments.
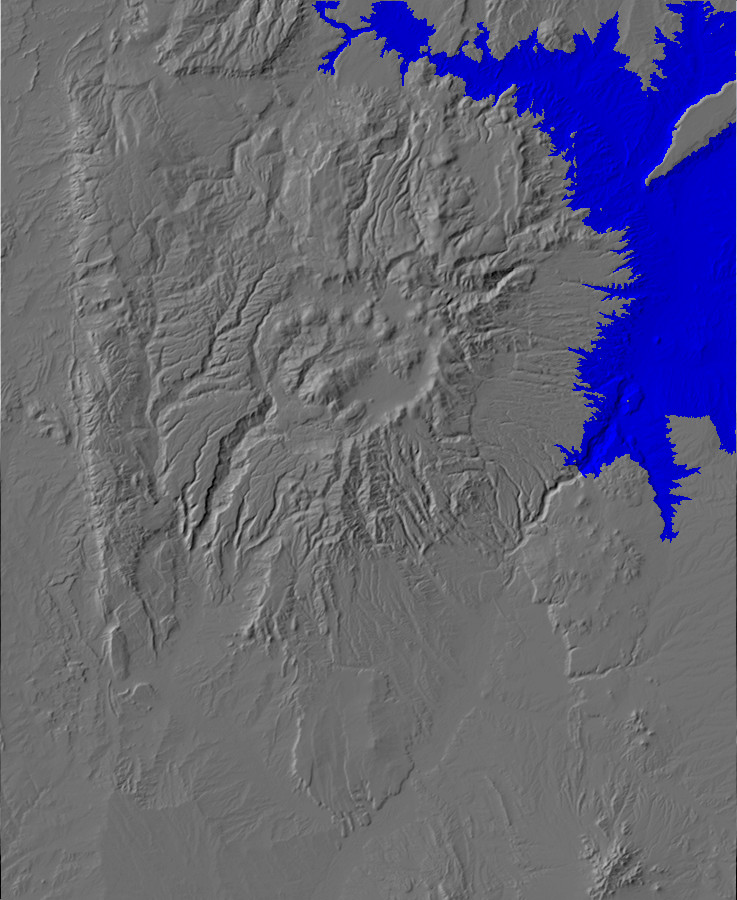
Relief map of the Jemez with approximate extent of
Culebra Lake highlighted in blue.
Lacustrine deposits are highly vulnerable to erosion, and few
traces remain in the Espanola Valley. One of the more dramatic
surviving exposures of lacustrine sediments is the diatomite mine
northwest of Espanola visible from Clara Peak.
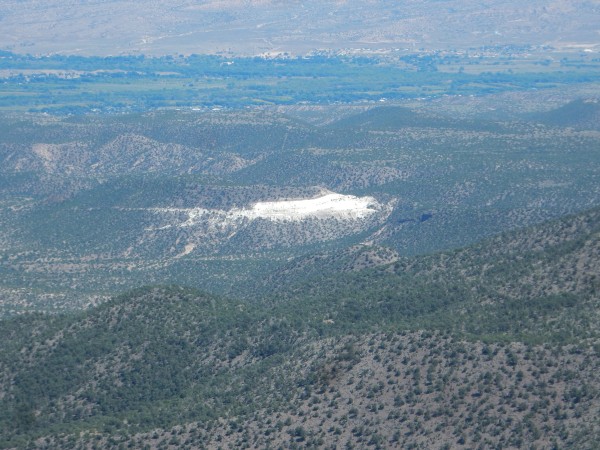
Diatomite mine. Lookine northeast from 36.035674N
106.24034W
The mine is operated by J.H. Rhoades Pumice Company. Diatomite,
also known as diatomaceous earth or as kieselguhr, is a
sediment consisting mostly of fossilized remains of diatoms.
Diatomite makes an excellent abrasive and is also used as a
filtering medium.
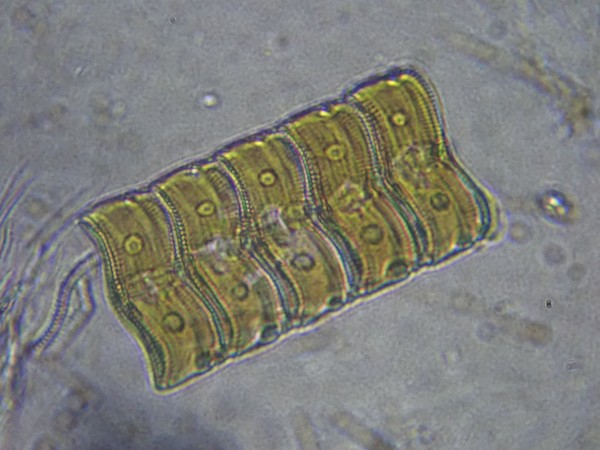
Freshwater Acnanthes diatoms from the author's
aquarium.
Diatoms are single-celled algae that form silica shells. They
first appeared in the fossil record around 200 million years ago,
and may have evolved to fill ecological niches opened by the
Permian-Triassic extinction. They subsequently became a major
component of plankton and may account for 20% of the Earth's
modern oxygen production. Diatoms flourish in both fresh and salt
water, and the diatoms here are freshwater diatoms, possibly from
Lake Culebra.
The Rio Grande had cut back down to within 130m (430') of its
current level at the time of the Toledo event, 1.6 million years
ago. Exposures of the Otowi Member are present on the east side of
the river north
of Cochiti, but the resulting dam must have been
short-lived, and the river rapidly cut down to its former channel.
The failure of this natural dam may account for deposits of Otowi
Member-rich clasts far downriver, at Socorro and Las Cruces.
Another short-lived dam was formed shortly afterwards by an
eruption of basaltic andesite that overlies the Otowi Member.
There is a shallow paleocanyon east of the river in this area that
shows the river course was temporarily diverted.
The river was down to 120m (390') above its current level when
the Valles event took place, 1.25 million years ago. At this time,
the canyon was much narrower than it is today, with a maximum
width of perhaps 600m (2000') and no evidence of the large slump
blocks seen today.
The Tsherige Member completely filled White Rock Canyon between
Chaquehi and Frijoles Canyon, and filled an eastern meander
of the canyon further north.
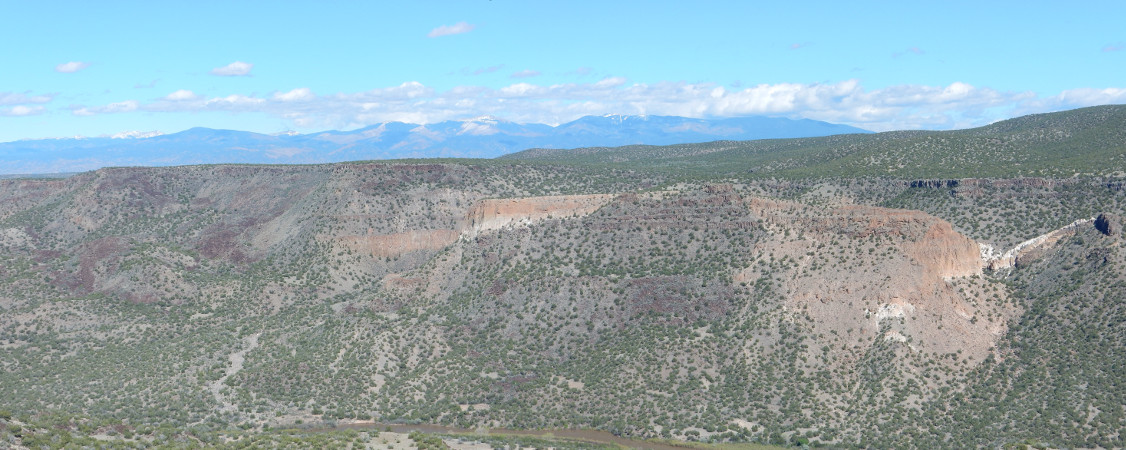
Tsherige Member filling eastern
meander in paleochannel of Rio Grande. Looking east from 35
47.367N 106 12.630W.
Here the beds of the Bandelier Tuff overlie a thin layer of river
gravel, which in turn lies atop Cerros del Rio basalts. From here
the paleocanyon turned sharply west, roughly along the modern
Water Canyon.
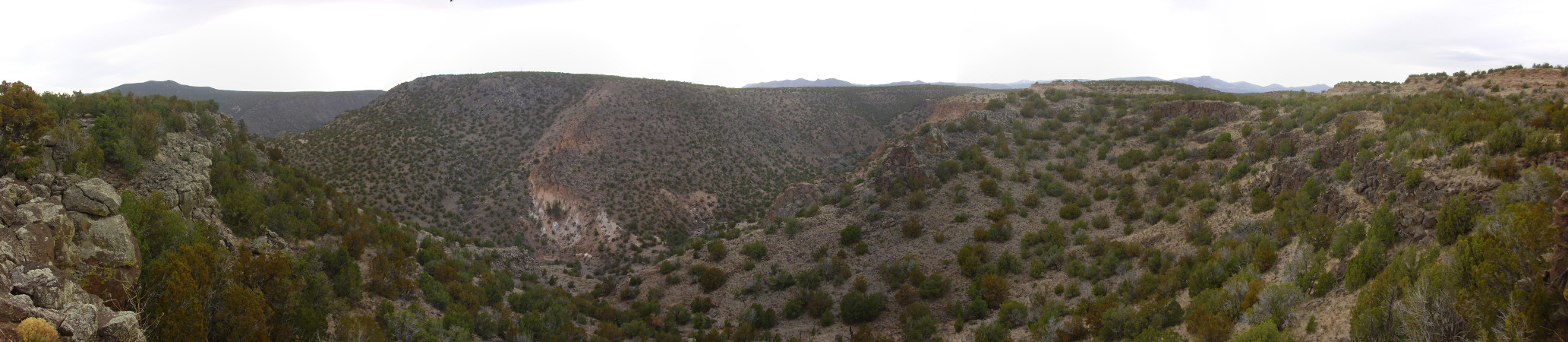
Cerros del Rio basalts in the confluence
of Water Canyon and Potrillo Canyon. 35
47.445N 106 12.712W
At far left and far right are the basalt cliffs that mark the
sudden drop from the relatively shallow Potrillo Canyon into the
much deeper Water Canyon. Water Canyon descends from the Pajarito
Plateau at center right, and continues to its confluence with
White Rock Canyon at left. Here part of the east rim of White Rock
Canyon is visible, with Montoso Peak on the skyline.
The large outcropping of light pink Bandelier Tuff in the south
wall of Water Canyon marks the westward meander of the
paleocanyon. This outcropping has not slumped down the
canyon wall; it was this thick when deposited 1.25 million years
ago. Since older Cerros del Rio basalt and underlying Santa Fe
Group sediments form the rest of the canyon wall, this shows that
the Bandelier Tuff filled a deep westward meander of the river.
This meander also left an outcrop of Bandelier Tuff on the north
wall of the canyon.
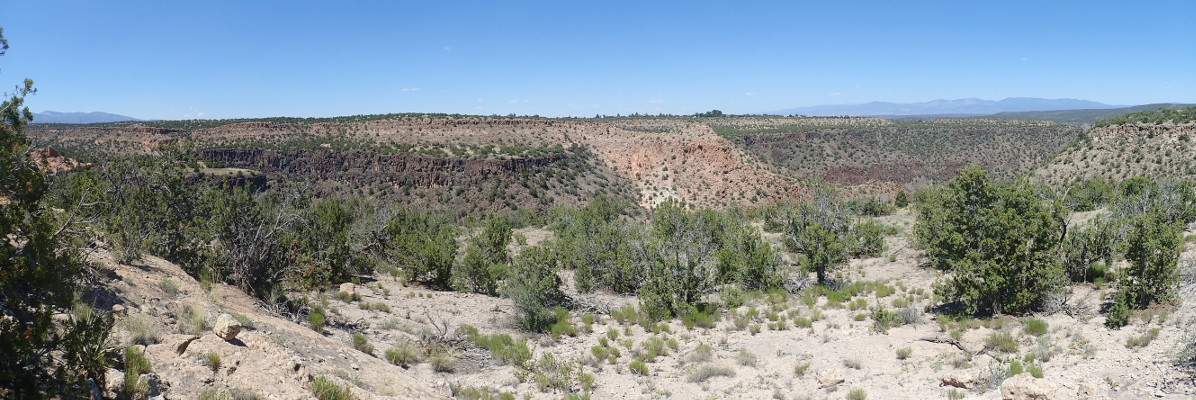
Banderlier Tuff filling meander in White Rock
paleocanyon exposed on north wall of Water Canyon. 35
47.578N 106 13.840W
The paleocanyon continues south along an unnamed tributary to
Ancho Canyon, where the modern canyon runs almost parallel to the
paleocanyon and exposes a great length of thick Tsherige Member
beds.
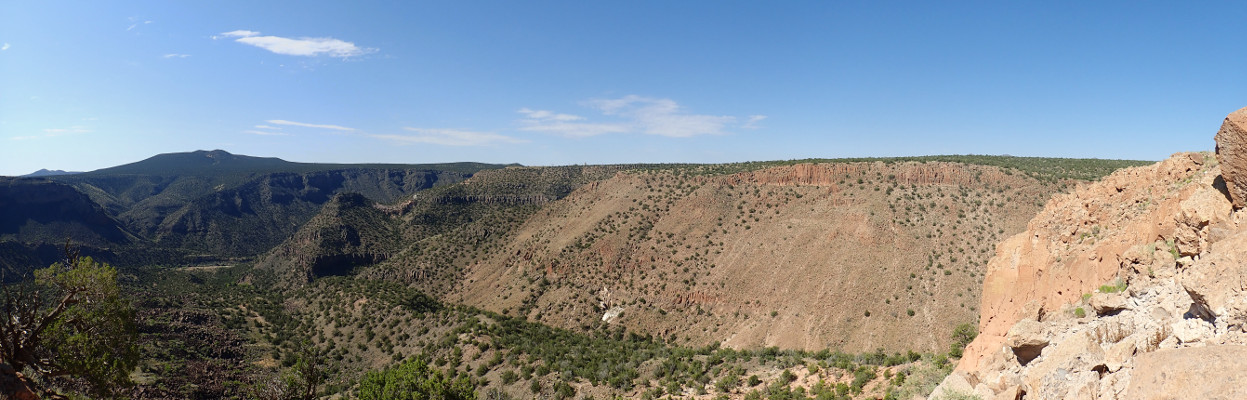
Banderlier Tuff filling meander in White Rock
paleocanyon in unnamed tributary to Ancho Canyon. 35.783458N
106.225969W
The paleocanyon is prominent in lower Frijoles Canyon.
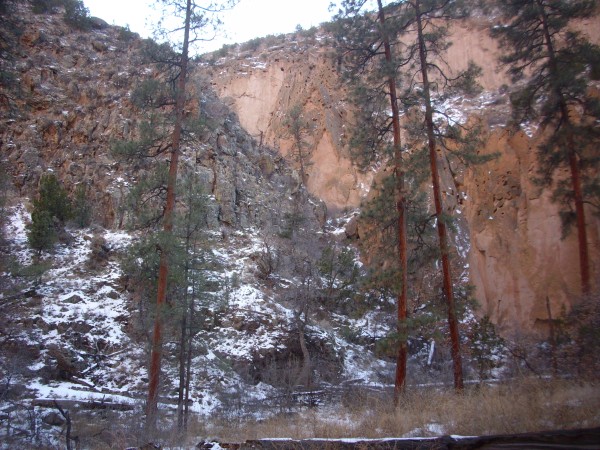
Contact between Cerros del Rio and Bandelier Formations
in lower Frijoles Canyon. 35
45.888N 106 15.661W
The damming of the Rio Grande near Frijoles Canyon raised the
river level to the highest since its beginnings in the Miocene, at
325m (1070') above its current level. The Rio Grande was forced 2
km (1.25 miles) east of its former course, and while it likely
rapidly cut through the tuff, it then encountered 200m (660') of
solid basalt that would have taken significantly longer to cut
through. Thus a second great Culebra Lake was formed that extended
perhaps 70 km (45 miles) to the north.This likely formed the lake
bars on the White Rock Canyon rim near Overlook Park.
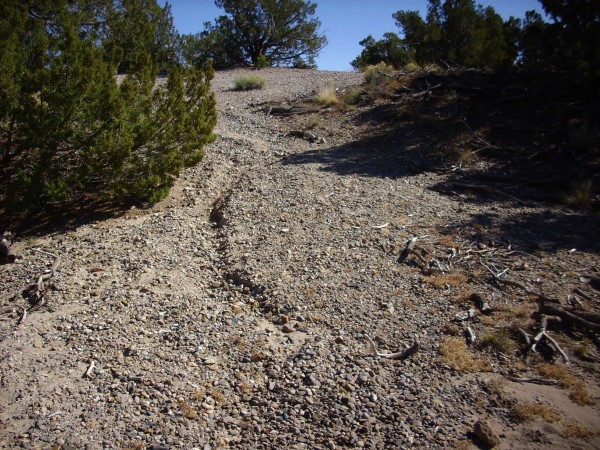
Gravel bank. 35
49.404N 106 11.057W
There are scattered beds of similar gravel for at least a mile
further down the canyon rim, and there is also a considerable
quantity of this gravel on a landslide block east of this point,
halfway down the canyon rim. The gravels beds appear to overlie
remnants of the Guaje Pumice and thus must be younger than 1.62
million years in age. The dam produced by the Tsherige Member is
estimated to have been 100m (180') higher than the canyon rim
where these cobbles are located.
The river was still probably around 150m (490') above its modern
level 0.62 million years ago. This is based on the location of ash
beds from the great Lava Creek eruption in Yellowstone at that
date, which spread ash across the United States. The rate of
erosion has been particular great in the last 100,000 years or so,
at about 50 cm (20") per thousand years. White Rock Canyon is now
about 250m (820') deep near the White Rock Overlook. The rate of
incision probably increased significantly sometime between 0.7 and
0.3 million years ago, when the San Luis Basin of southern
Colorado became integrated into the Rio Grande watershed, more
that doubling the watershed area above White Rock Canyon. This was
also about the time the upper and lower reaches of the Rio Grande
finally became integrated in the El Paso area. (Prior to then the
Rio Grande ended in a series of lakes in northern Mexico.) The
final integration of the Rio Grande dramatically dropped the base
level of the upper Rio Grande, which had continued to slowly
deposit sediments in the northern Rio Grande Rift up to that time.
Thereafter rapid erosion set in, producing most of the badlands
seen along the northern Rio Grande today.
There have been several smaller lakes over the last 70,000 years,
mostly created by a large slump near Water Canyon that has
repeatedly slid into the river channel during periods of unusually
wet climate.The last such lake is thought to have formed about
12,400 years ago, close to the start of the Holocene.
Zeolite ledges
There are a number of prominent ledges eroded into the Bandelier
Tuff in Ancho Canyon.
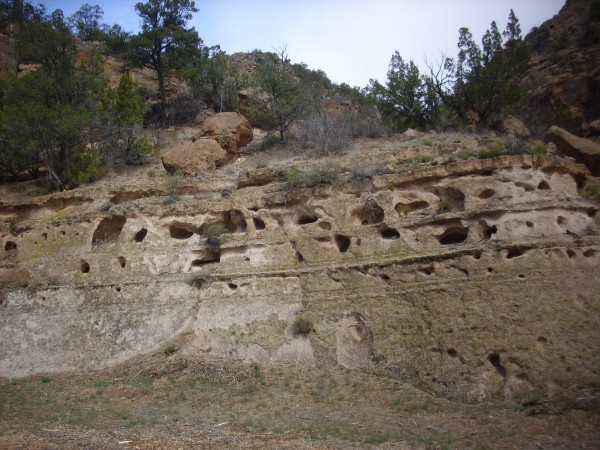
Zeolitized ledges in Tsherige Member. 35
47.344N 106 15.836W
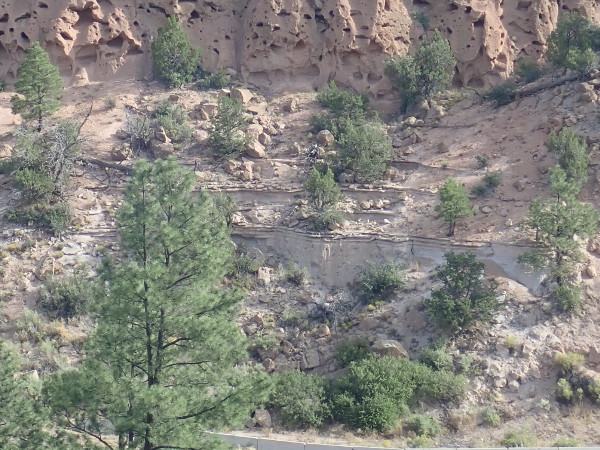
Zeolitized ledges in Tsherige Member. Looking north from
35.7872955N
106.2587791W
The ledges do not correspond to any flow boundaries or other
bedding features in the tuff, and they can be traced into other
canyons along the Pajarito Plateau. They are believed to show lake
levels in nearby White Rock Canyon. The lake water would have
saturated the tuff up to the lake level, and the few inches just
above the water level would have produced an ideal environment for
zeolites to form in the tuff. These are hydrous silicate
minerals that fill pore spaces and increase the durability of the
tuff. Radiometric analysis of the zeolite crystals gives an age of
about 1 million years for the ledges, but this age is rather
uncertain.
The Sierra Ladrones
Formation
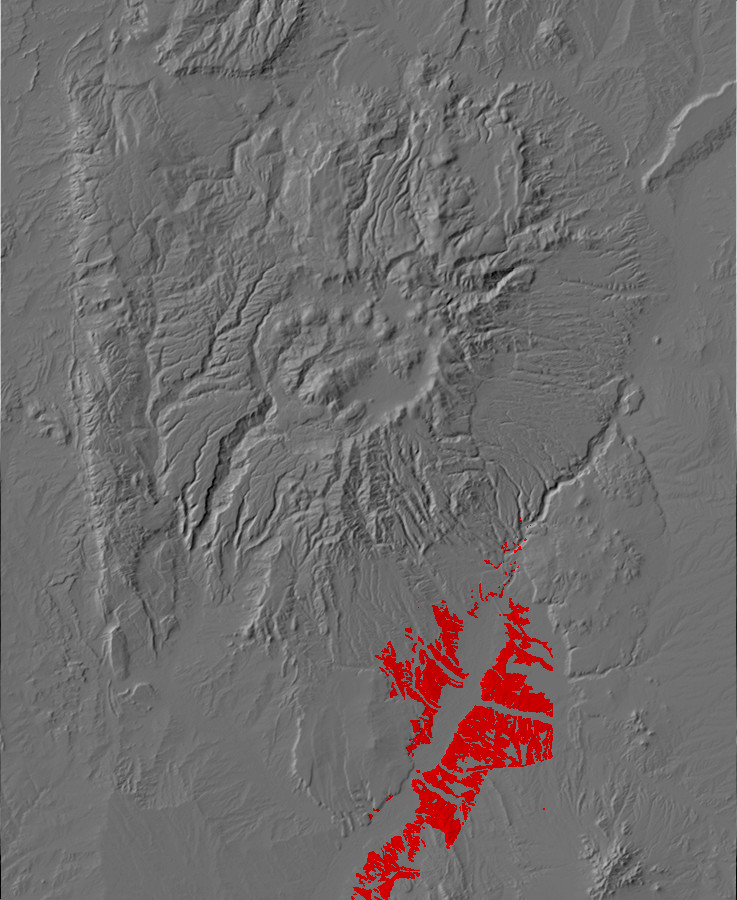
Relief map of the Jemez with Sierra Ladrones Formation
outcroppings highlighted in red
Deposits interpreted as old river gravel are present throughout
the Cochiti area. These river gravels cannot be dated directly,
but some beds are overlain further north by formations known to be
about 2.5 million years old, showing that parts of the old river
gravel must be older than this. The older portions of these gravel
beds were mapped as "old gravels" in the classic Smith and Bailey
map of the Jemez Mountains, but they have since been assigned to
the Sierra Ladrones Formation of the upper Santa Fe Group.
Excellent outcrops of the Sierra Ladrones Formation can be found
south
of the small town of Pena Blanca. This photograph shows the
sandstone facies of the formation.
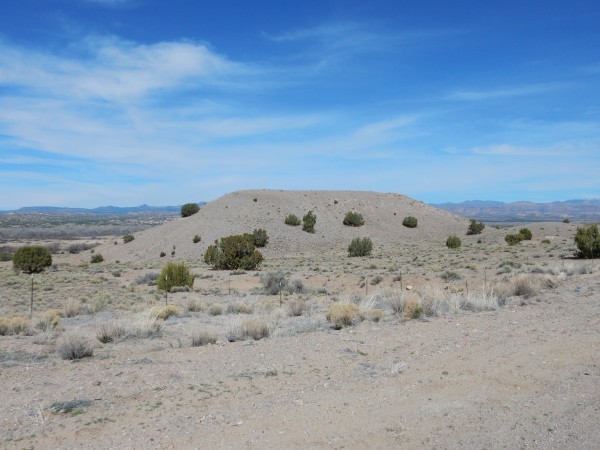
Sandstone facies, Sierra
Ladrones Formation. 35.543532N
106.3457787W
Here a fault has thrown up a hill of the underlying axial gravel
facies of the formation.
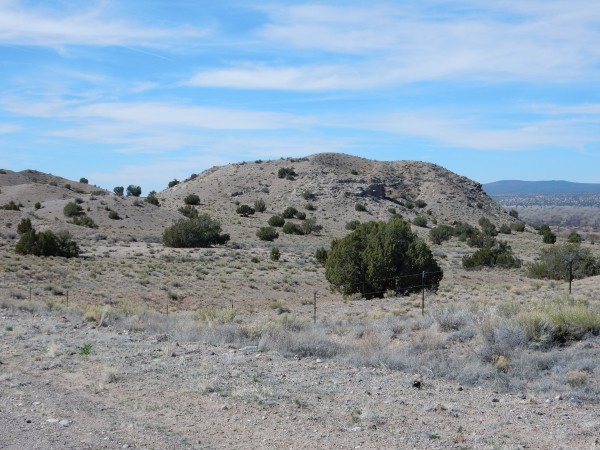
Axial gravel facies, Sierra Ladrones Formation. 35.543532N
106.3457787W
The San Francisco Fault passes through the notch at left. The
terrain on the near side of the notch is more of the sandstone
facies, while the hill on the other side is the axial gravel
facies. Axial gravel is laid down in the central channel of a
river, in this case the ancestral Rio Grande. These beds are
difficult to date precisely but cannot be older than about 6.5
million years or much younger than 1.6 million years, based on the
ages of the individual rock fragments deposited as gravel in the
formation and its relation with volcanic formations in the area.
Further down the road, a very pretty road cut.
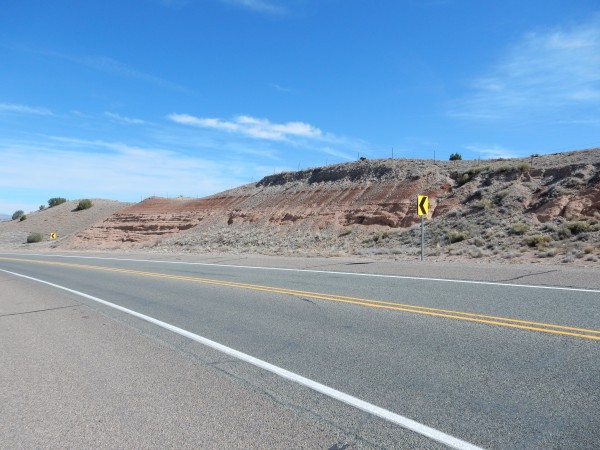
Road cut in piedmont faces of
Sierra Ladrones Formation. 35.503778N
106.3394288W
The nicely bedded red sediments are the piedmont facies of the
Sierra Ladrones Formation. This is sediment brought in by the
Santa Fe River and Galisteo Creek from the east. East of this
location, this facies contains abundant fossils of early horses (Equus)
and ancient relatives of elephants (Gomphotherium).
The gravel on top is relatively young alluvial gravel. The lower
portions of the gravel contain volcanic ash that has been traced
to the Lava Creek eruption of the Yellowstone caldera, 630,000
years ago, and presumably this gravel is about that age.
The gravel itself contains a mix of quartzite clasts, probably
Precambrian quartzite from the Tusas Mountains to the north, and
basalt clasts, likely from the Cerros del Rio southeast of White
Rock. This means this gravel was brought in by the Rio Grande.
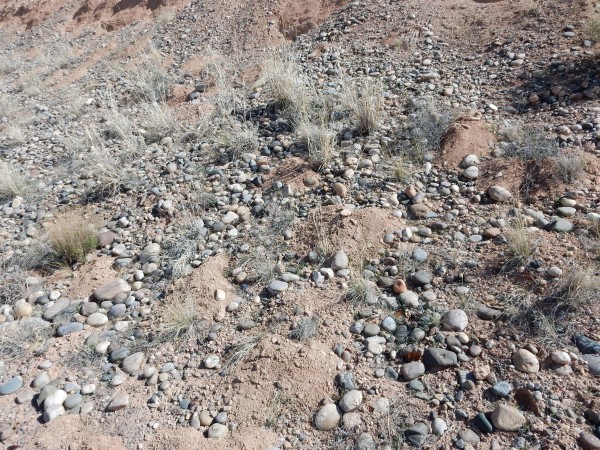
Terrace grave atop Sierra
Ladrones Formation. 35.503778N
106.3394288W
The Sierra Ladrones Formation continues far to the south; indeed,
its type section is in the Socorro area in southern New Mexico. It
is well exposed along I-25 just south of San Felipe Pueblo, at the
southern limit of the Jemez region.
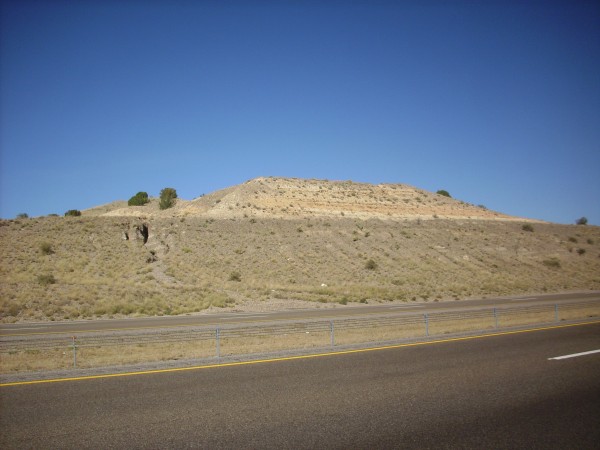
Silt and gravel beds of the
Sierra Ladrones Formation. 35.4121877N
106.4169554W
Here we see light tan silt beds atop a thick bed of coarse
gravel. The gravel is axial gravel of the ancestral Rio Grande
while the silt is likely overbank deposits, deposited
after the main channel had shifted elsewhere when the river
overlowed its banks onto its floodplain.
Terrace gravels
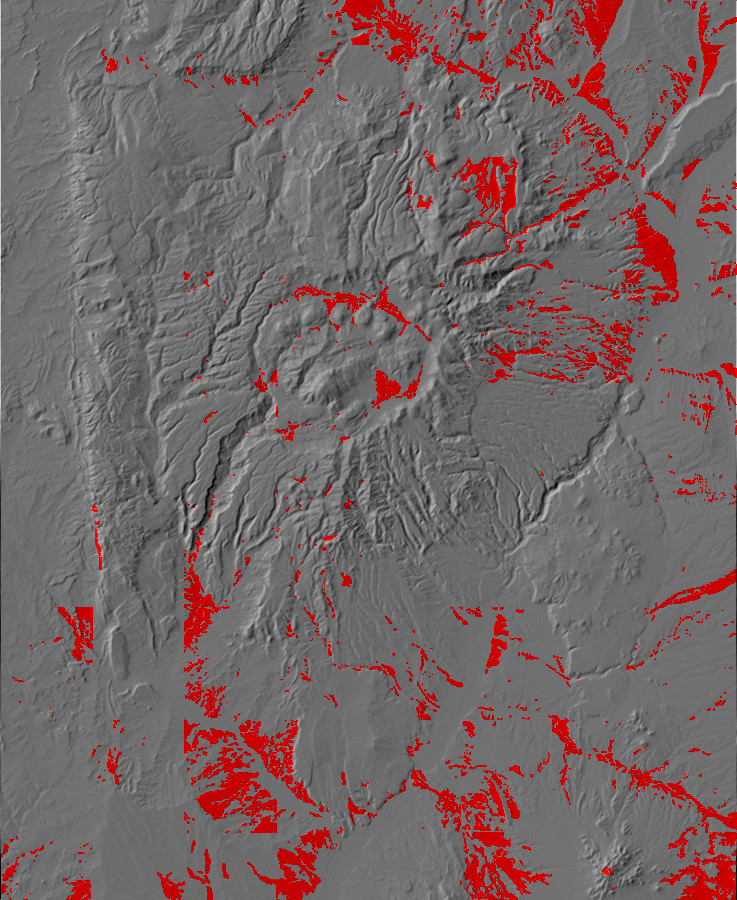
Relief map of the Jemez with terrace gravels highlighted
in red.
The ultimate fate of material eroded from the Jemez Mountains is
to be carried down the Rio Grande River to the Gulf of Mexico.
However, only fine clay is likely to make the trip directly,
suspended in the water. Sand and small pebbles roll and bounce
along the river bottom, a mode of transport called saltation.
Larger clasts work their way down river channels in several steps,
likely driven by infrequent but powerful flood stages of the
river.
The ability of a river to carry sediment depends on how swift the
river is. Turbulent motions in rapidly moving water keep the
smallest particles in suspension. Swift flow at the river bottom
keeps sand and pebbles rolling and bouncing. When the river
changes speed, the carrying capacity also changes. Thus, when a
river slows, it tends to drop sediments, a process called aggradation.
Since the swiftness of a river is largely determined by how level
its bed is, a river descending a steep slope quickly cuts down (incises)
a deep channel, while a river that is crossing nearly level ground
reaches an equilibrium in which aggradation balances erosion.
For an ancient river emptying into the sea, such as the
Mississippi, the effect is that the river cuts a very broad flood
plain of very low relief and elevation. The flood plain is
typically underlain by very fine overbank sediments while the
river channel has coarser sand and pebbles. Since sediment tends
to be deposited on the inside of curves in the river, and eroded
from the outside of curves, curves tend to grow and the river
becomes a meandering river, with a single channel showing
intricate loops. At the other extreme, very young rivers flowing
across steep ground tend to form several channels that split and
reconnect to form a braided river.
Curiously enough, some of the best examples of meandering streams
in the Jemez area are within the caldera itself, where the flat
caldera floor provides low relief for the East Fork Jemez River
and San Antonio Creek, which strongly meander. The Rio Grande in
the Espanola Basin is young enough, geologically speaking, that it
is intermediate between a braided river and a meandering river,
showing incipient meandering in some stretches and braided
features in others.
A river in an area of recent uplift, such as the Rio Grande in
New Mexico, may have relatively level stretches ending in knickpoints,
where the river loses elevation relatively rapidly as it crosses
resistant beds or other features than hinder rapid downcutting.
The knickpoint is said to establish the base level for the
river upstream, which becomes the level of a floodplain around the
river if the knickpoint lasts long enough. When the knickpoint is
cut through, or if some other process causes the river to begin
cutting downwards again, it is said to have abandoned its
former floodplain, and the remnants of that floodplain may remain
as terraces.
Northern New Mexico has experienced regional uplift for at least
the last several million years, and the larger rivers have
experienced repeated drops in their base levels. This has produced
multiple terrace levels along major rivers, such as
the Rio Grande. These terraces are often covered
with terrace gravels, deposited by the main channel of the river
as it cut a flat surface onto the underlying bedrock.
The youngest terraces are obvious geomorphic features.
Geomorphology is the study of how the modern topography of the
land is dictated by the underlying geology. A volcanic cone is an
example of an geomorphic feature; the cone-shaped mountain is a
direct result of a specific geologic process. Hogback ridges are
another example, formed by erosion that levels nearby ground but
leaves a set of resistant rock beds as a ridge. A young terrace is
distinctive flat ground away from the main flood plain of a river.
Here is an example.
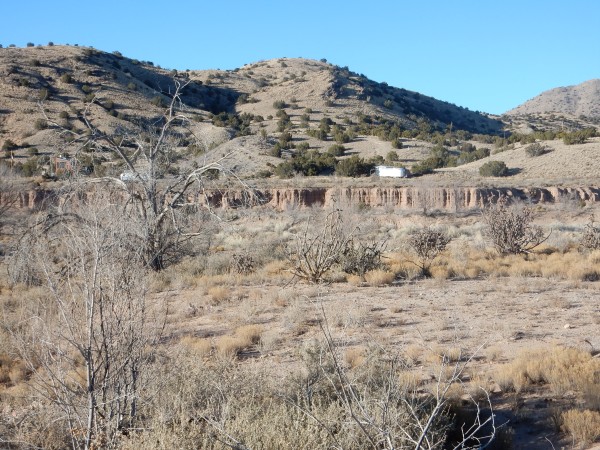
Young terrace near Galisteo Creek. Looking north from 35
26.341N 106 07.354W
The low ground is the current channel and associated (small)
floodplain of a small tributary of Galisteo Creek. The flat ground
on the near and far sides are young river terraces, showing where
a sudden drop in the base level for Galisteo Creek caused the
river to abandon its previous floodplain and cut downwards to
establish the current floodplain. This is the youngest of six such
terrace levels in the Galisteo Creek drainage, which record
repeated drops in base level.
Older terraces are eroded to where they no longer are an obvious
geomorphic feature. They are identified instead from the pattern
of coarse gravel resting on a flat surface cut in older bedrock
near a river. There are good examples of contacts between terrace
gravels and the underlying Abiquiu Formation west of Abiquiu. Here
the terrace gravels are derived from red source rocks, making a
stark contrast with the white Abiquiu Formation.
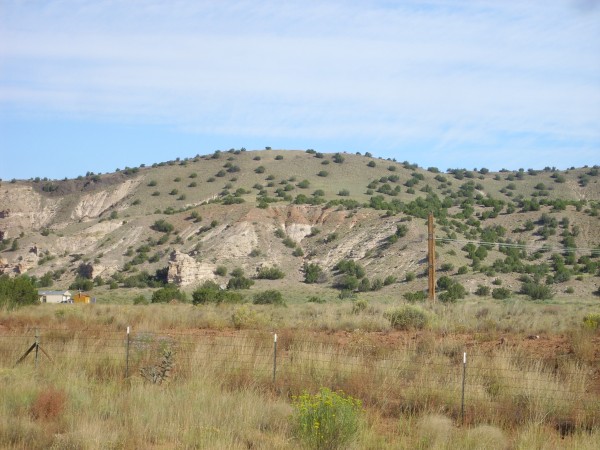
Terrace gravel on Abiquiu Formation. Looking northwest
from 36
12.751N 106 20.507W
The terrace gravel is the red bed about halfway up the hill side.
The white beds beneath and in back are Abiquiu Formation. The
terrace gravel thus is perched on the side of the hill, at what
was once the level of a side channel of the ancestral Rio Chama.
Terrace gravels associated with the ancestral Rio Chama extend
further south, and are well-exposed along Highway 84 between
Abiquiu and Medanales. Here the gravels form low hills west of the
highway.
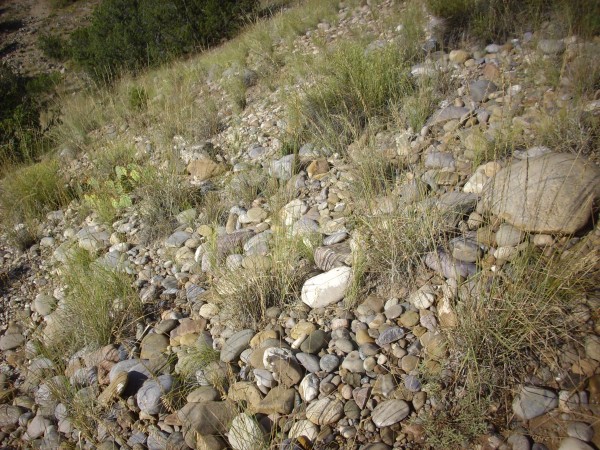
Terrace gravel. 36
11.809N 106 14.396W
Terrace gravels are found in the area south of Black Mesa, with a
particular well-defined contact with the Chamita Formation.
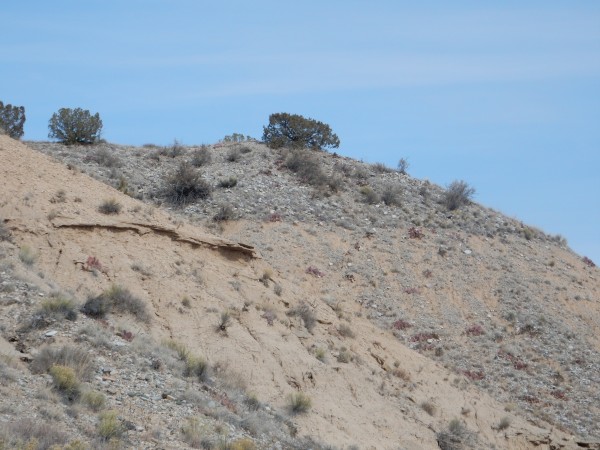
Terrace gravels over Chamita Formation. 36
06.997N 106 03.469W
This is the younger of two terrace levels identified along the
Rio Grande in this area, with an age of around 140,000 years.
The terrace gravels are also well-exposed along Thirtyone Mile
Road west of Espanola.
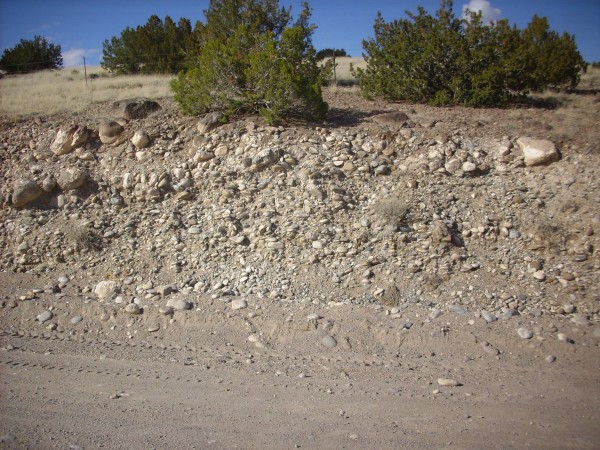
Upper terrace gravel along Thirtyone Mile Road. 36
00.659N 106 07.021W
Nearby the underlying Santa Fe Group sediments are exposed.
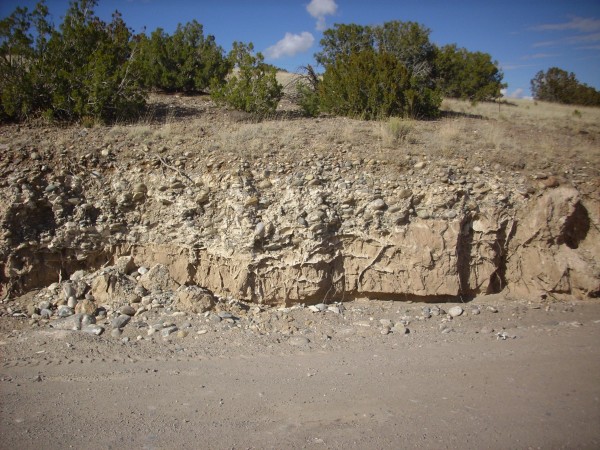
Upper terrace gravel along Thirtyone Mile Road. 36
00.659N 106 07.011W
The large, well-rounded clasts show that this deposit is an axial
deposit, representing the major river channel in this area. The
geologic map for this area identifies this as terrace deposits of
the Rio Chama-Rio Grande confluence, with an estimated age of
350-650 thousand years. The age is based in part on the presence
of Lava Creek B ash beds near the base of the deposit. The deposit
in the second photograph has been heavily calichified.
There are beds of terrace gravel along the main highway to Los
Alamos near the point where it crosses the Rio Grande.
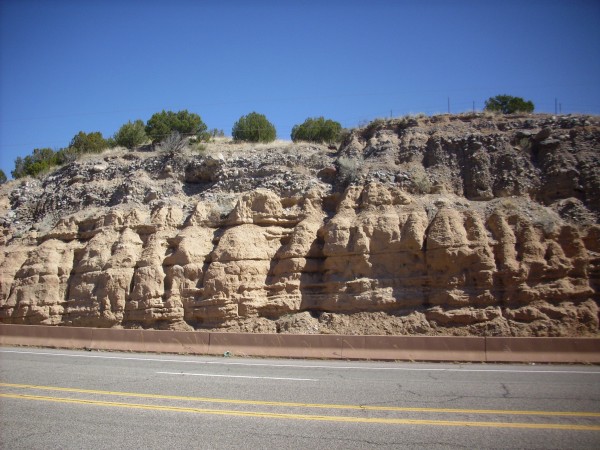
Terrace gravel on top of Santa Fe Formation. 35.878N
106.144W
These can be difficult to distinguish from the Totavi Lentil of
the Puye Formation, but their location close to the river, without
any overlying beds, hints at their true nature. The Totavi Lentil
has, in fact, been interpreted as terrace gravels from successive
stands of the Rio Grande as it cut downward and shifted eastward
due to the growth of the Jemez volcanic field. These were
subsequently buried by fanglomerates of the Puye Formation.
There are some particularly fine examples of terrace gravels near
Cochiti Dam. One example is so well sorted, with a very distinct
upper contact, that my first thought was that this was artificial,
placed by highway workers in the road cuts for erosion control or
some other purpose. It was not until I saw the beds exposed in
arroyos well away from the road that I was convinced they were a
natural geologic feature.
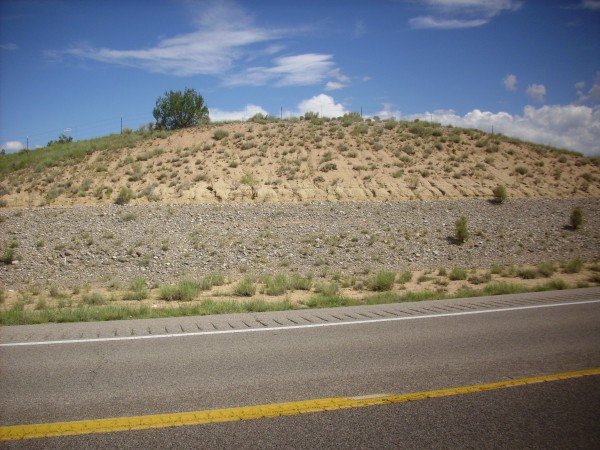
Old gravel in Cochiti area. 35
36.406N 106 19.681W
This is one of the younger terrace gravels in this area, probably
less than 100,000 years old. It is at the fourth of five distinct
terrace levels that have been mapped in the area. The silty, tan
beds above the gravel are mapped as eolium, which is silt and fine
sand brought in largely by the wind.
Similar old gravels are found in the Abiquiu area and probably
represent gravel deposited by the ancestral Rio Chama.
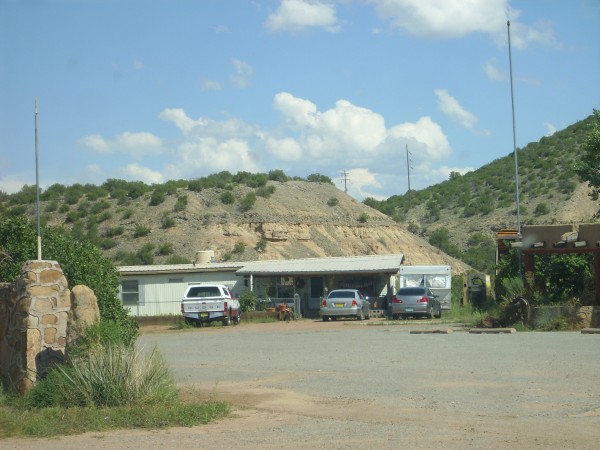
Old gravel in Abiquiu area. 36
12.749N 106 16.916W\
This is apparently an old axial gravel of the Rio Chama,
deposited on Chama-El Rito Member, Tesuque Formation. The flat
ground above has ruins of an Ancestral Puebloan city, Poshuinge.
Some chapters back, we saw that there is a distinctive bed of
gravel between the Tesuque Formation and overlying terrace gravels
throughout the area west and north of Espanola. This marker bed is
particularly well exposed at Arroyo Largo.
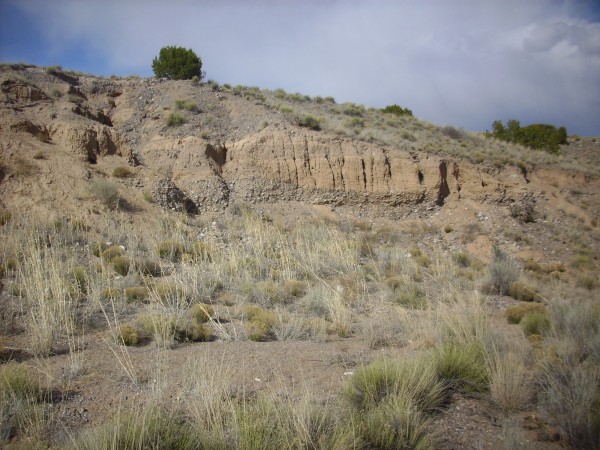
Quartzite gravel marker bed. 36
01.457N 106 05.527W
This bed is very distinctive, consisting of well rounded clasts
of quartzite typical of the Tusas Mountains to the north. It mark
a geologically brief interval sometime in the last two million
years when the ancestral Rio Grande and its tributaries in the
Espanola area carried a heavy load of gravel from the mountains to
the north. One wonders if it correlates with the beds we've just
seen at Abiquiu and Cochiti and, if so, what event it records.
Alluvium
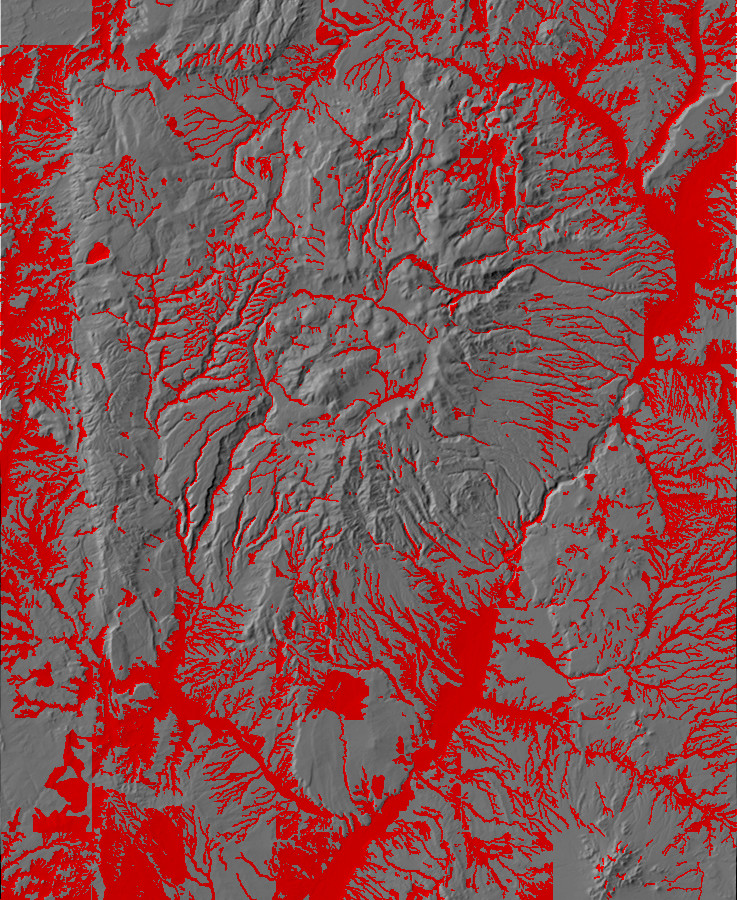
Relief map of the Jemez with alluvium highlighted in
red.
Finally, we reach the modern flood plains, where alluvium is
being transported today or has been transported in the recent
past. River channels and their floodplains are among the more
obvious and recognizable of land forms. Alluvium often fills
canyon and arroyo bottoms even where there is no permanent stream.
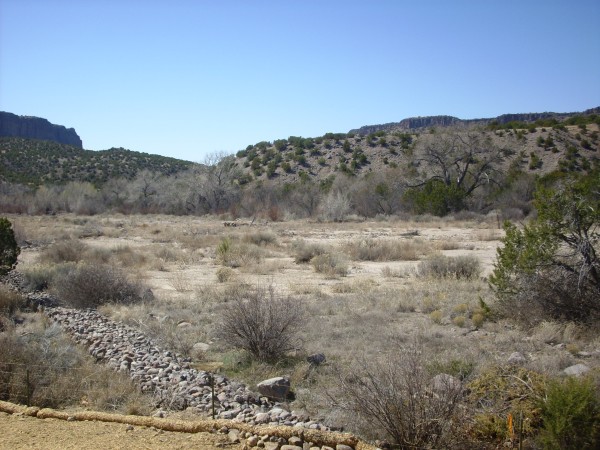
Alluvium near confluence of Pueblo Canyon with Rio Grande.
35
52.546N 106 08.681W
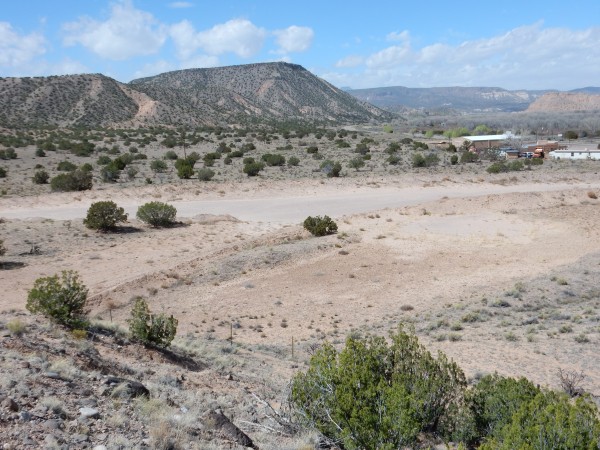
Arroyo west of Poshuouinge ruins. 36
12.740N 106 16.665W
Arroyos such as this one can become very wet indeed during heavy
rain. With their extremely flat sandy bottoms, they’re an
important land form in the Jemez area.
Many arroyos in the Jemez Area are characterized by very steep
banks, subject to occasional rockfalls.
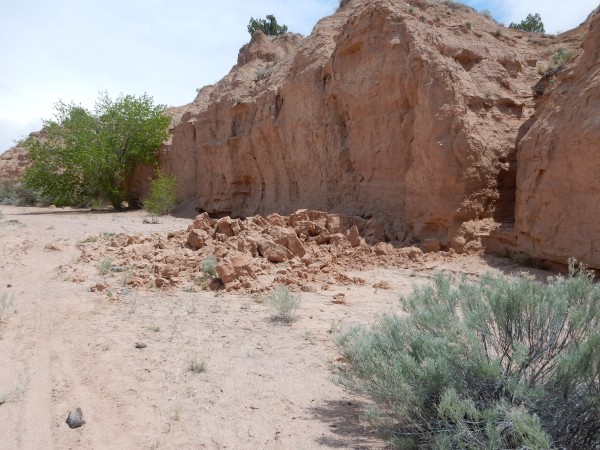
Rockfall along arroyo near Buckman. 35
50.239N 106 09.134W
Here the occasional flash flood has undercut the banks, leaving
them unstable against collapse.
Some of the most impressive alluvium deposits are associated with
the valles of the Valles caldera. These are broad valleys
winding among the ring domes that are devoid of timber but support
lush grass. We've seen many photographs of these areas in previous
chapters.
The lack of timber arises from two causes. First, the valles are
floored with thick beds of clay in which trees have considerable
difficulty putting down roots. Second, the winter climate in the
valles can be brutal.
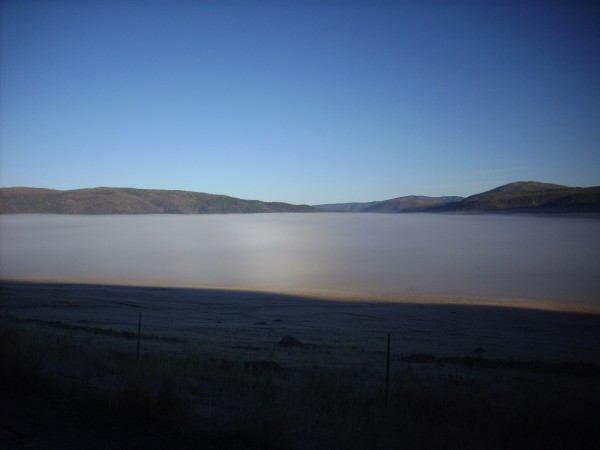
Valle Grande fogged in. 35
51.103N 106 27.312W
This is a photograph of Valle Grande, the largest of the valles,
on an October morning. The layer of fog is not unusual. The
caldera accumulates cold air, producing a temperature inversion
that often leads to the formation of fog or a low cloud layer.
During the winter months, when there can be long stretches of dry
and sunny weather, the snow cover evaporates off the valles floors
and leaves tree seedlings exposed to bitter night cold. This is
more than they can take.
Alluvium is also found along river channels, but here there is a
delicate balance between deposition and erosion. Where fire has
stripped cover and increased the power of water, erosion can
predominate, as in the areas burned by the 2011 Las Conchas fire.
Here the channel is being rapidly cut downwards to produce
extensive gullies.
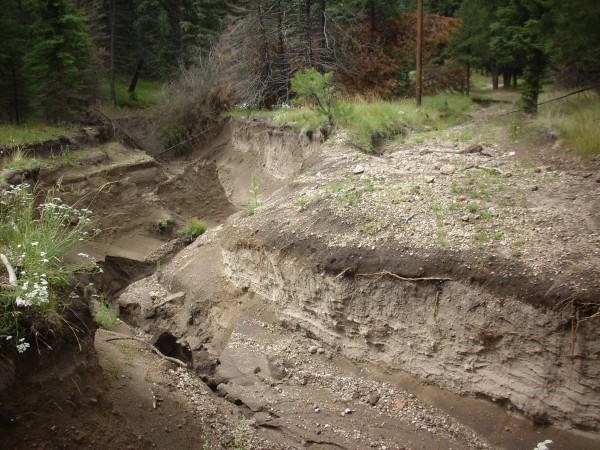
Some real gullies. 35.835N
106.546W
They’re real, and they’re spectacular. This section was at least
ten feet deep, and it cut right across the old jeep road I was
following. I had to work upstream a significant distance to find a
spot where I could cross.
These channels represent concentrated flow. If the watershed of
such a channel was a square kilometer, and erosion in the
watershed averaged just a centimeter per thousand years, this
would correspond to a cube of debris more than two meters on a
side moving down the channel each year. Most likely the rate is
much lower in ordinary years and much higher in years following a
fire or other disturbance. There is evidence that the canyon
bottoms of the Pajarito Plateau experienced a burst of alluvium
deposition between 12,000 and 8000 years ago, based on carbon
dating of charcoal fragments. A borehole in Ancho Canyon found a
floodplain deposit 10,000 years old five meters (16.5') below the
present canyon floor.
Sheetwash
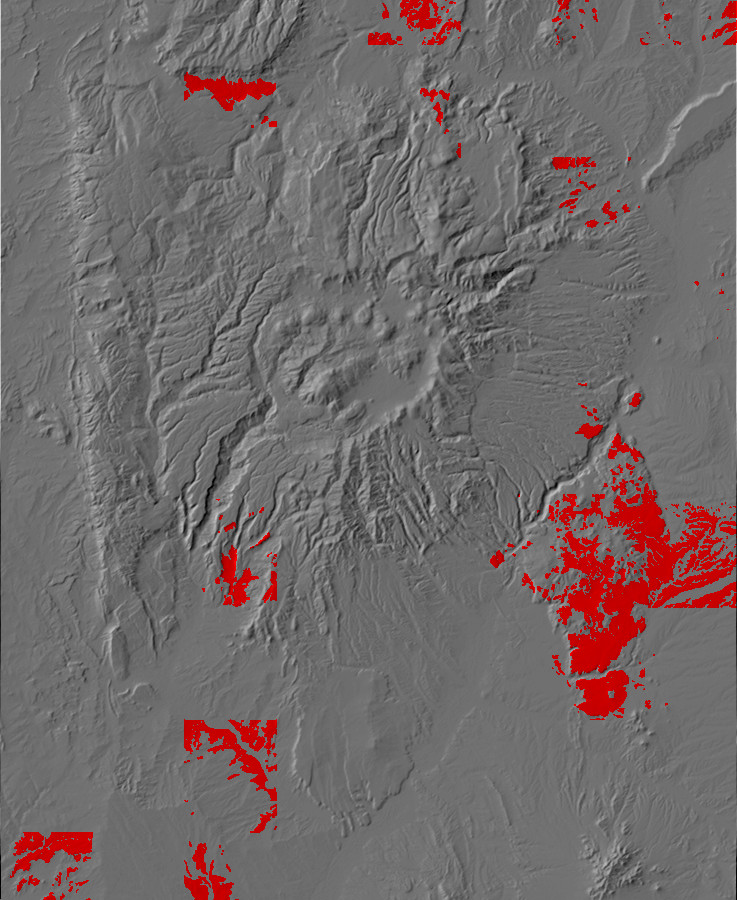
Relief map of the Jemez with sheetwash highlighted in
red.
Alluvium tends to be concentrated in river channels, but, in arid
climates, areas of low relief may become covered with sheetwash.
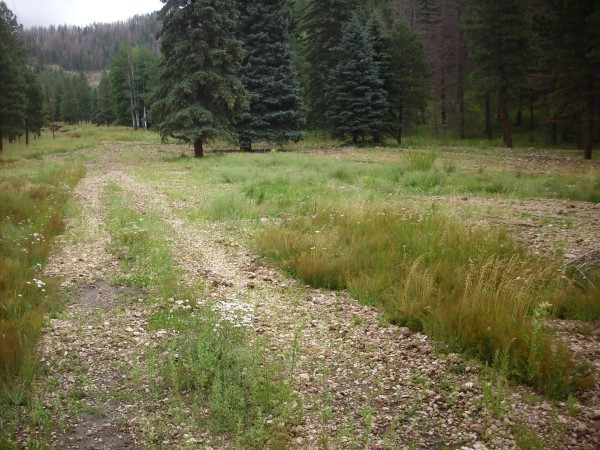
Sheetwash deposits on valley floor. 35
50.118N 106 32.746W
Sheetwash is deposited where flood waters run across a large
surface without being channeled. It is distinct from a floodplain,
formed by regular seasonal overflow of a river from its channel.
Sheetwash deposits occur where there is no nearby river channel.
Our story has now reached the point where it becomes our
story. In the next chapter, Homo sapiens arrives
on the scene.
Next page: Emerging from deep time
Copyright © 2015 Kent G. Budge. All rights reserved.